增益值与功率换算公式
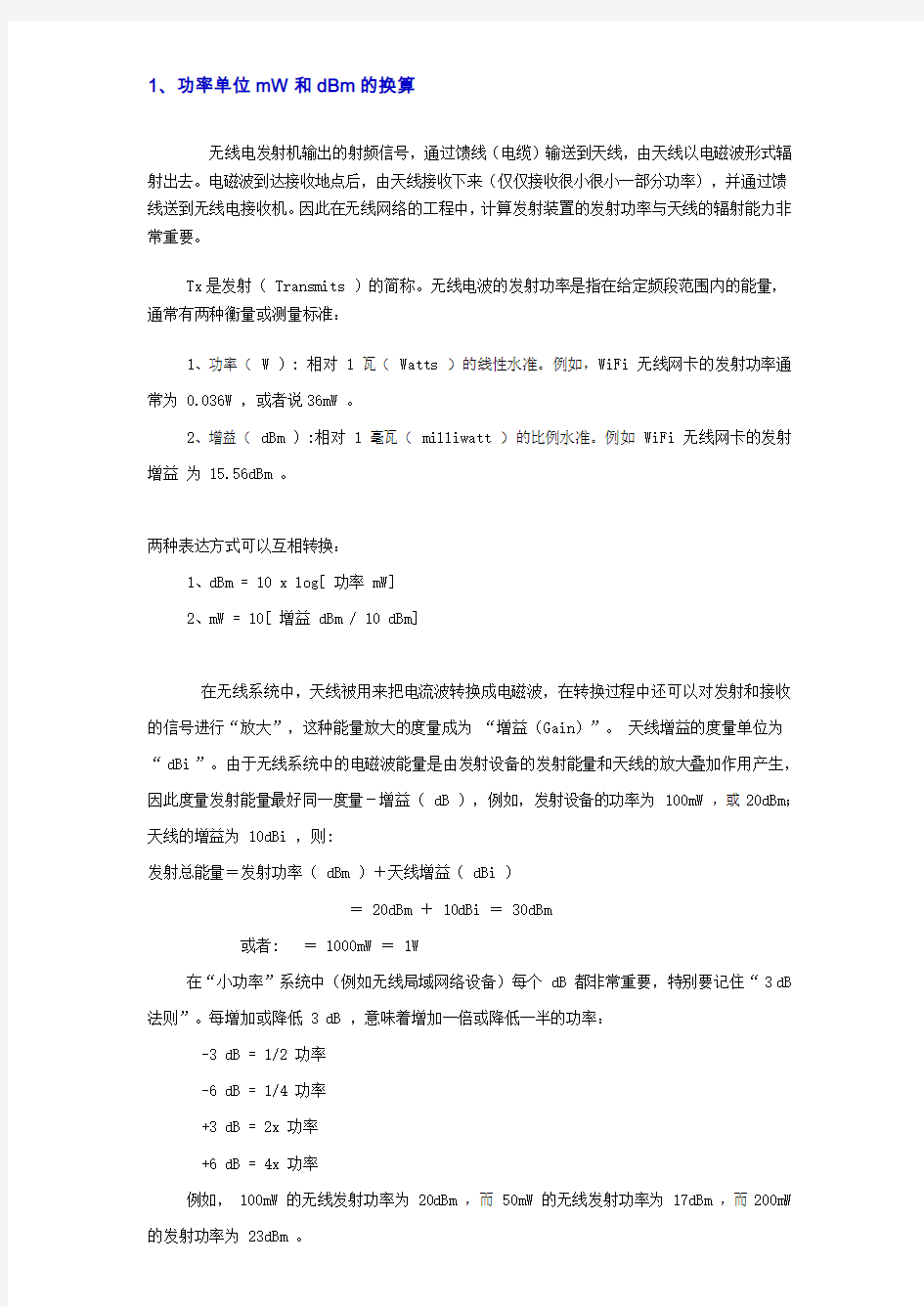
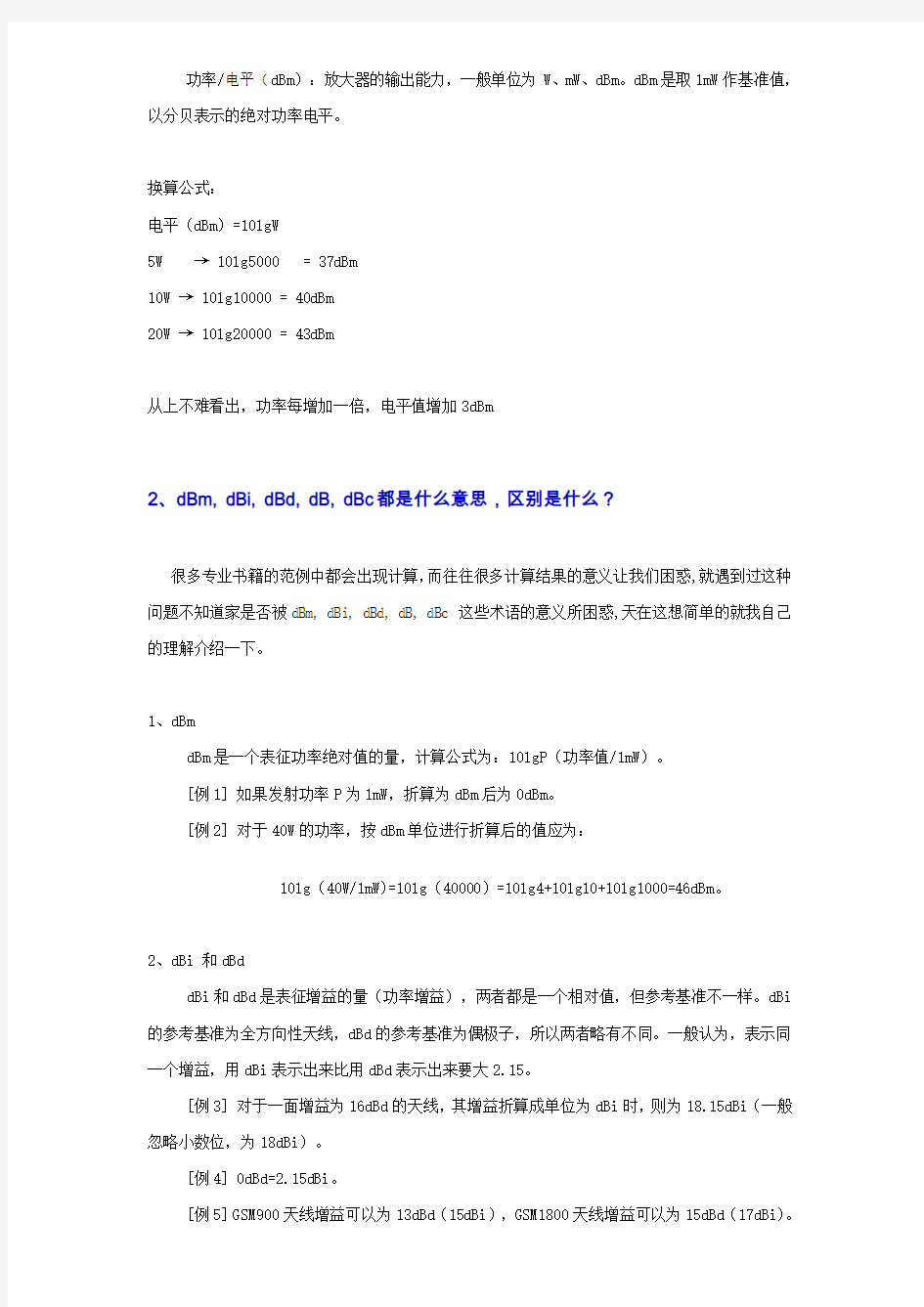
1、功率单位mW和dBm的换算
无线电发射机输出的射频信号,通过馈线(电缆)输送到天线,由天线以电磁波形式辐射出去。电磁波到达接收地点后,由天线接收下来(仅仅接收很小很小一部分功率),并通过馈线送到无线电接收机。因此在无线网络的工程中,计算发射装置的发射功率与天线的辐射能力非常重要。
Tx是发射( Transmits )的简称。无线电波的发射功率是指在给定频段范围内的能量,通常有两种衡量或测量标准:
1、功率( W ): 相对 1 瓦( Watts )的线性水准。例如,WiFi 无线网卡的发射功率通常为 0.036W ,或者说36mW 。
2、增益( dBm ):相对 1 毫瓦( milliwatt )的比例水准。例如 WiFi 无线网卡的发射增益为 15.56dBm 。
两种表达方式可以互相转换:
1、dBm = 10 x log[ 功率 mW]
2、mW = 10[ 增益 dBm / 10 dBm]
在无线系统中,天线被用来把电流波转换成电磁波,在转换过程中还可以对发射和接收的信号进行“放大”,这种能量放大的度量成为“增益(Gain)”。天线增益的度量单位为“ dBi ”。由于无线系统中的电磁波能量是由发射设备的发射能量和天线的放大叠加作用产生,因此度量发射能量最好同一度量-增益( dB ),例如,发射设备的功率为 100mW ,或20dBm;天线的增益为 10dBi ,则:
发射总能量=发射功率( dBm )+天线增益( dBi )
= 20dBm + 10dBi = 30dBm
或者: = 1000mW = 1W
在“小功率”系统中(例如无线局域网络设备)每个 dB 都非常重要,特别要记住“ 3 dB 法则”。每增加或降低 3 dB ,意味着增加一倍或降低一半的功率:
-3 dB = 1/2 功率
-6 dB = 1/4 功率
+3 dB = 2x 功率
+6 dB = 4x 功率
例如, 100mW 的无线发射功率为 20dBm ,而 50mW 的无线发射功率为 17dBm ,而200mW 的发射功率为 23dBm 。
功率/电平(dBm):放大器的输出能力,一般单位为W、mW、dBm。dBm是取1mW作基准值,
以分贝表示的绝对功率电平。
换算公式:
电平(dBm)=10lgW
5W → 10lg5000 = 37dBm
10W → 10lg10000 = 40dBm
20W → 10lg20000 = 43dBm
从上不难看出,功率每增加一倍,电平值增加3dBm
2、dBm, dBi, dBd, dB, dBc都是什么意思,区别是什么?
很多专业书籍的范例中都会出现计算,而往往很多计算结果的意义让我们困惑,就遇到过这种问题不知道家是否被dBm, dBi, dBd, dB, dBc这些术语的意义所困惑,天在这想简单的就我自己的理解介绍一下。
1、dBm
dBm是一个表征功率绝对值的量,计算公式为:10lgP(功率值/1mW)。
[例1] 如果发射功率P为1mW,折算为dBm后为0dBm。
[例2] 对于40W的功率,按dBm单位进行折算后的值应为:
10lg(40W/1mW)=10lg(40000)=10lg4+10lg10+10lg1000=46dBm。
2、dBi 和dBd
dBi和dBd是表征增益的量(功率增益),两者都是一个相对值,但参考基准不一样。dBi 的参考基准为全方向性天线,dBd的参考基准为偶极子,所以两者略有不同。一般认为,表示同一个增益,用dBi表示出来比用dBd表示出来要大2.15。
[例3] 对于一面增益为16dBd的天线,其增益折算成单位为dBi时,则为18.15dBi(一般忽略小数位,为18dBi)。
[例4] 0dBd=2.15dBi。
[例5] GSM900天线增益可以为13dBd(15dBi),GSM1800天线增益可以为15dBd(17dBi)。
3、dB
dB是一个表征相对值的量,当考虑甲的功率相比于乙功率大或小多少个dB时,按下面计算公式:10lg(甲功率/乙功率)
[例6] 甲功率比乙功率大一倍,那么10lg(甲功率/乙功率)=10lg2=3dB。也就是说,甲的功率比乙的功率大3dB,则功率大一倍。
[例7] 7/8 英寸GSM900馈线的100米传输损耗约为3.9dB。
[例8] 如果甲的功率为46dBm,乙的功率为40dBm,则可以说,甲比乙大6dB。
[例9] 如果甲天线为12dBd,乙天线为14dBd,可以说甲比乙小2 dB。
4、dBc
有时也会看到dBc,它也是一个表示功率相对值的单位,与dB的计算方法完全一样。一般来说,dBc 是相对于载波(Carrier)功率而言,在许多情况下,用来度量与载波功率的相对值,如用来度量干扰(同频干扰、互调干扰、交调干扰、带外干扰等)以及耦合、杂散等的相对量值。在采用dBc的地方,原则上也可以使用dB替代。
I F1Q . V CC ?2V BE;on
.1 ?a.R2 . a2R1e V in=V T
; .2.
V out;cascode . V CC ?2V BE;on ?a2R1I F1Q e V in=V T : .3.
I F1 . I0e
.V BE.V in
.=V T . I F1Q e V in=V T ;
Monolithic broadband transimpedance ampli?ers and their high frequency small and large signal characteristics using CBE-based GaInP/GaAs HBT technology
Jae-Woo Park a, Saeed Mohammadi a, Dimitris Pavlidis a,*, Christian Dua b, Jean-
Luc Guyaux b, Jean-Charles Garcia b
a Department of EECS, University of Michigan, 1301 Beal Avenue, Ann Arbor, MI 48109-2122, USA
b Thomson-CSF, Central Research Laboratory, Domaine de Corbeville, 91404 Orsay, France
Received 14 January 2000; accepted 30 March 2000
Abstract
Monolithic broadband transimpedance ampli?ers were developed using chemical beam epitaxy (CBE) based GaInP/
GaAs HBT technology. The developed HBTs showed a cut-o. frequency (f T) of 60 GHz and a maximum oscillation
frequency (f max) of 100 GHz using laterally etched undercut (LEU) technology. The fabricated ampli?ers had a maximum bandwidth of 19 GHz and an associated transimpedance gain of 47 dBX. The small and large signal characteristics of two transimpedance ampli?er designs with similar gain were investigated. The cascode design when
compared to the common-emitter design, provided a higher bandwidth and a more open eye diagram, as well as less
sensitive to input power gain and eye diagram pattern. ó 2000 Published by Elsevier Science Ltd. All rights reserved.
1. Introduction
High speed and high capacity optical transmission
systems require high-speed sensitive current input preampli
?ers to condition the input signal received in the
form of current pulses from PIN diodes or avalanche
photodiode detectors (APDs). Transimpedance feedback
ampli?ers are commonly used as preampli?ers with
low-input impedance and ˉat-gain characteristics. Considerations
made in the development of the transimpedance
ampli?ers include process yield which is related
to cost reduction, bandwidth, gain ˉatness, sensitivity to
input power, performance stability and device lifetime
under actual circumstances [1].
Future optical transmission systems will operate at
bit rates up to 40 Gbs?1 and higher and silicon devices
that are traditionally used in these designs are no longer
suitable for such speeds. Compound semiconductor devices
have been studied extensively for such applications
and provide not only a higher bandwidth of operation,
but they also o.er possibility of integration with photodiode.
FETs were the ?rst compound semiconductor
devices used for photoreceiver applications [2,3]. State of
the art photoreceivers with 27 GHz bandwidth have
been demonstrated using InAlAs/InGaAs HFETs [4].
Transimpedance ampli?ers have also been demonstrated
using heterojunction bipolar transistors (HBTs) [5±7].
Monolithic integration, higher reliability and simple
processing technology of GaAs based HBTs in addition
to their improved linearity and noise performance are
some of the advantages for the application of these devices
in high-speed optical communication systems. Al-
GaAs/GaAs HBT preampli?ers based on advanced p.
regrown extrinsic technology have demonstrated excellent
performance (34.6 GHz) with a transimpedance
gain of 41.6 dBX [8]. GaInP/GaAs HBT monolithic
integrated circuits have also been used for optical communication
systems at bit rates up to 10 Gbs?1 [9].
GaInP/GaAs HBTs are well known alternatives over
AlGaAs/GaAs HBTs due to their attractive features
Solid-State Electronics 44 (2000) 2059±2067
* Corresponding author. Tel.: +1-734-647-1778; fax: +1-734-
763-9324.
E-mail address: pavlidis@https://www.360docs.net/doc/fa2205748.html, (D. Pavlidis).
0038-1101/00/$ - see front matter ó 2000 Published by Elsevier Science Ltd. All rights reserved. PII: S0 03 8 -1 10 1 (00 )0 0 07 4 -5
such as excellent etching selectivity which contributes to
process yield, device reliability [10] and improved carrier
injection e?ciency [11,12] due to their large valence
band discontinuity. GaInP/GaAs HBT monolithic
transimpedance ampli?ers can be used for short distance
communication (0.8 lm) such as board-to-board or
chip-to-chip data links but also as an alternative solution
to InP-based technology for long distance communication
(1:3±1:5 lm) by means of ˉip chip hybrid
mounted InP photodiodes.
A ?rst report on GaInP/GaAs HBT technology and
its use for high-speed transimpedance ampli?ers with bandwidth exceeding 19 GHz was recently presented by
the authors [13]. This paper provides further details on
the design, fabrication and high frequency characteristics
of transimpedance ampli?er OEICs using the
GaInP/GaAs HBT approach. Both small and large signal characteristics are reported. The large-signal characteristics
of these ampli?ers become an important issue
when the front-end preampli?er receives large power
signals from a photodiode as often as it occurs in optical receiver systems [14]. Advanced systems of this type, use
a variable gain preampli?er to establish a linear regime
of operation [1]. Another alternative is the use of a
simple high gain preampli?er as a limiting ampli?er. In
this case, the ampli?er operates in large-signal regime
which can a.ect its speed and output signal waveform
and consequently the bit-error-rate performance of the
optical receiver system. Section 2 of the paper presents
HBT design consideration and the process technology applied to device and circuit fabrication. Section 3 presents the design and experimental characteristics of transimpedance ampli?ers using this technology. The
large-signal properties of the ampli?ers are reported in Sections 4 and 5.
2. HBT design and process technology
In this work, the single GaInP/GaAs HBTs were
optimized in terms of microwave performance by evaluating an appropriate layer structure and process. A
GaInP tunneling emitter barrier design was chosen,
since the tunneling barrier acts as a ``mass ?lter'' and provides a much higher ratio of tunneling probabilities,
a higher emitter injection e?ciency, and high transconductance compared with traditional designs leading
therefore to an improved microwave performance. The
thin tunneling barrier was sandwiched between the
n-type emitter and p-type base of an otherwise homojunction bipolar transistor design. Details of this tunneling
HBT design were reported earlier by the authors
[15].
The GaInP/GaAs HBTs were fabricated on layers
which were grown by a specially developed hydride and hydrogen-free chemical beam epitaxy (CBE) process
which guaranteed a very high degree of reproducibility
of growth parameters with small defect content and high output capability [16]. The process starts with Ti/Pt/Au
non-alloyed metal deposition for emitter contacts followed
by an etch of InGaAs, GaAs emitter caps, and
GaInP emitter. A short separation of 0.1 lm between
the self-aligned base and the emitter assures small base resistance and hence improved maximum oscillation frequency. The base metal consists of Pt/Ti/Pt/Au and
was deposited on a 600 _A thick GaAs base. The process
was followed by etching the GaAs base and collector
regions and then deposition of Ti/Pt/Au for collector contact. Laterally etched undercut (LEU) was developed
and applied to the collector region to further reduce
base±collector capacitance (C BC) while avoiding base resistance degradation. The collector layer under the
base contact was removed for this purpose. Fig. 1 shows
the SEM cross-section pictures of the fabricated HBT
(a) without and (b) with LEU technology. The 2 _ 30
lm2 single emitter HBTs were measured using a network analyzer from 0.5 to 25.5 GHz. A reduction of C BC from
46.7 to 36.5 fF by lateral etching of about 6000 _A leads
to 10% gain improvement at 10 GHz as shown in Fig. 2
and a higher maximum oscillation frequency. The most commonly used approach for minimizing C BC is based
on ion-implantation through the active base layer. The
Fig. 1. Cross section of the device with laterally etched undercut technique.
2060 J.-W. Park et al. / Solid-State Electronics 44 (2000) 2059±2067 process analyzed here is based on LEU of the collector
layer and o.ers therefore simplicity and a very e.ective
way of reducing C BC.
The key in this technology step is the incorporation
of a thin (1 _ 1019 cm?3 doped) GaInP layer between the
n. and n?GaAs collector which provides selectivity in
etching and determines the etching pro?le below the
base. The 2 _ 30 lm2 single emitter HBTs fabricated
using LEU technology demonstrated an f T of 58 GHz
and f max of 100 GHz at I C . 20 mA, V CE . 2:5 V. This corresponds to an f T increase of 11% and f max increase
of 25% as a result of using LEU technology as shown in
Fig. 2. The microwave performance of the investigated GaInP/GaAs HBTs shows only a slight change with bias
and therefore permits robust circuit design. The HBTs showed a thermal resistance of 1430 KW
?1 as explained
in Section 3.
The technology used to fabricate the monolithic integrated circuits employs additional steps of Ni±Cr thin
metal ?lm deposition for monolithic resistors and Al2O3
?lm deposition as the insulator for high-Q MIM
monolithic capacitors. A SiO2 PECVD deposited layer
was used as the ?nal step for HBT passivation and was
found to induce very little degradation to the HBT characteristics.
3. Design and experimental performance of the transimpedance
ampli?ers
The 2 _ 30 lm2 single emitter HBT was modeled
using small and large-signal equivalent circuit models developed by the authors which were introduced in HP EESOF LIBRA and HSPICE [17,18]. The small-signal
high-frequency model is based on physical parameters
and is extracted directly from cold and hot S-parameter measurement [17]. The large-signal model is based on Gummel±Poon equivalent circuit and includes a thermal resistance that accounts for device heating [18]. The temperature e.ect is observed under high dissipated
power conditions when the junction temperature rise manifests itself with a negative slope in I C±V CE characteristics. The large-signal model combines the smallsignal equivalent circuit with data obtained from DC
parameters extracted from Gummel-plots and I C±V CE characteristics. This model also accounts for parasitic reactances due to device interconnects. Both small and
large-signal HBT equivalent circuits were developed
within HP-EESOF LIBRA environment for compatibility with high-frequency circuit simulation needs.
Circuit design was carried-out using models for HBTs, resistors and capacitors in di.erent LIBRA environments such as linear, DC, large signal S-parameters and
harmonic-balanced test benches.
The inset of Fig. 3(a) shows the schematic of the
common-emitter based transimpedance ampli?er (design
A) employed in this work. The input signal current
is ampli?ed by the common emitter stage of transistor
T1 and resistor R1. The feedback network which consists
of resistors R2 and R3 stabilizes the transimpedance
gain. Transistor T2 serves as a bu.er to isolate the
feedback network from the gain stage. T3 and R4 behave
as another bu.er to isolate the feedback network
and output. The inset of Fig. 3(b) shows the schematic
of another transimpedance ampli?er (design B) which
was also explored and fabricated in the same lot. The schematic is identical to that of the simple transimpedance ampli?er of Fig. 3(a) except that in this case, a
cascode topology is used as the gain stage. As shown in
the following, the cascode design not only improves the bandwidth, but also contributes to better large signal performance.
Fig. 3(a) also shows the measured small-signal transmission coe?cient (S21) and transimpedance gain of
the common-emitter based transimpedance ampli?er measured with an HP 8722D network analyzer. A S21
gain of 17 dB with a bandwidth of 10 GHz has been measured using on-wafer probe measurement. Fig. 3(b) shows S21 and transimpedance gain of the cascode based
transimpedance ampli?er. A similar S21 gain of 17 dB
with a bandwidth of 15 GHz has been achieved for bias condition A .V CC . 5 V). The transimpedance gain was
47 dBX and 50 dBX for designs A and B, respectively.
By increasing the collector voltage of the cascode stage
(bias condition B, V CC . 7 V), the bandwidth could be increased to 19 GHz while the associated S21 and transimpedance gain were 12 dB and 47 dBX, respectively.
Fig. 4 shows a photograph of the fabricated transimpedance circuit (design A). Coplanar waveguide transmission
lines were employed to connect the ampli?er to
50 X terminations. The chip size for the circuits was
1125 _ 800 lm2.
Fig. 2. Microwave performance of 2 _ 30 lm2 single emitter
HBT with and without LEU technology at V CE . 2:5 V, I C . 20
mA.
J.-W. Park et al. / Solid-State Electronics 44 (2000) 2059±2067 2061 4. Large signal analysis
To show the e.ect of input signal on DC biasing of
ampli?ers, we have ?rst performed a comparison of transimpedance ampli?er characteristics based on analytical expressions and a simple T-equivalent circuit for
HBTs. This analysis was done at small frequencies (DC
to 1 GHz) for which the junction reactances are negligible
so that an estimate of circuit performance can be
made on a DC basis without signi?cant impact from
reactive elements. Fig. 5(a) shows the circuit schematics
of the cascode transimpedance ampli?er (design B) using
the HBT T-equivalent circuit. The emitter current of the
input transistor (T1) can be written as
I F1 . I0e
.V BE.V in
.=V T . I F1Q e V in=V T ; .1.
where I F1 is the emitter current of T1, I F1Q is the quiescent component of the emitter current, I0 is the emitter
±base dark current, a is the HBT emitter injection e?ciency, V BE is the quiescent component of the base±
emitter voltage, V in is a sinusoidal input voltage and V T is
the thermal voltage. For the purpose of the analysis, it is assumed that all HBTs in the circuit have the same
current gain since they are all operating around the same
bias condition. One can ?nd the quiescent current
component I F1Q and the output voltage V out for this
circuit as
I F1Q . V CC ?2V BE;on
.1 ?a.R2 . a2R1e V in=V T
; .2.
V out;cascode . V CC ?2V BE;on ?a2R1I F1Q e V in=V T : .3.
Therefore, the DC component of the output voltage can
be approximated by the average of minimum and
maximum voltage of the output signal with 90° and 270°phase shift, respectively with respect to the input. By employing the same approach, one can also establish a
DC large-signal model for the common-emitter transimpedance ampli?er (design A). The output voltage, in
this case, is given by the following equation.
Fig. 3. Transmission coe?cient S21 and transimpedance gain of
(a) design A and (b) design B vs. frequency. The schematics are
also shown in the insets.
Fig. 4. Photograph of the fabricated transimpedance ampli?er. (Design A).
2062 J.-W. Park et al. / Solid-State Electronics 44 (2000) 2059±2067 V out;common-emitter . .V CC ?2V BE;on.
_
.1 ?a.R2
.1 ?a.R2 . aR1e V in=V T
: .4.
Based on the previously described theory, one can
estimate the change of the output voltage DC component
as the input voltage swing increases for the two
di.erent designs. Such a comparison is shown in Fig.
5(b), where the DC component of the output voltage is plotted vs. the amplitude of the sinusoidal input voltage. Design A which is based on the common-emitter approach shows a larger variation of the DC bias for the
output stage transistor T3 due to self-biasing. Self-biasing occurs when the non-linear operation of the transistor generates by recti?cation an additional DC
component which e.ectively changes the operating bias
of the transistor. Transistor HBT T2 of the previous
stage of design A also shows signi?cant self-biasing
while transistor HBT T2 of design B is not subjected to
signi?cant self-biasing.
To explain the di.erence in the output voltage DC component, one should notice that the two ampli?ers compared in this study were designed to provide similar gain. In common-emitter design (design A), HBT T1 has
a large voltage gain and therefore a large e.ective Miller capacitance is in parallel to resistor R1. In order to keep
the gain high, the value of R1 must be higher than that
of design B (cascode con?guration) in which the Miller
e.ect is minimal (R1 . 400 X in design A vs. R1 . 75 X
in design B). Since optimal bias needs to be employed
for large bandwidth of operation, the bias voltage of the circuit (V CC) has to increase to provide the same current
in resistor R1 (V CC . 12 V in design A vs. V CC . 5 V in
design B to provide 20 mA current in R1). The optimum collector±emitter voltage for the HBTs employed in the circuits is 3 V and transistor T1 of design A is biased at 3
V, while its maximum collector voltage can reach 12 V.
On the other hand, the minimum attainable collector
voltage of this transistor, neglecting the o.set voltage, is
close to 0 V. Therefore, at high input power, transistor
T1 operates between 0 and 12 V resulting in a shift of
collector voltage DC component to about 6 V. Design B, however, shows a smaller shift in DC components in presence of high input power due to smaller V CC, and
thus the self-biasing is not pronounced in this design;
under high power input signal conditions, the cascode
design corresponds to signal swings between 0 and 5 V.
Self-biasing e.ects can deteriorate the gain of the
ampli?er by driving the HBT out of its optimum bias setting. It manifests by a reduction of the forward transmission coe?cient S21, but may also result in pronounced input and output mismatching (higher S11 and
S22). Full large-signal S-parameter simulation was performed for this purpose in a second step of the analysis.
The transimpedance circuits were analyzed for this
purpose using the large-signal model developed by the authors for GaInP/GaAs HBTs [18]. Fig. 6(a) shows the change in S11, S21 and S22 parameters for the ampli?er of design A as the input incident power increases. The simulation is performed at three di.erent frequencies
to view the e.ect of HBT reactances on large signal Sparameters. The large signal forward transmission coe
?cient S21 reduces as the input power increases. This reduction is pronounced at smaller frequencies (2 and 5
GHz) where the HBT reactive elements such as C BC and
C BE are negligible. The input signal passes, in this case, primarily through nonlinear elements of the HBT such
as base±emitter diode and the gain varies in a non-linear fashion with the input power level. At higher frequencies
Fig. 5. (a) T-equivalent circuit of cascode transimpedance
ampli?er. (b) DC component of the output voltage as a function
of input voltage, simulated using T-equivalent large-signal
model of the HBT.
J.-W. Park et al. / Solid-State Electronics 44 (2000) 2059±2067 2063 (8 GHz), a signi?cant part of the signal passes through reactive elements (C BC and C BE) which are linear by a
?rst degree approximation. Therefore, the ampli?er itself behaves more linearly resulting in less variation of
the large-signal S21 parameter. The large-signal input
and output reˉection coe?cients S11 and S22 also depend
upon input power as shown in Fig. 6(a). Large-signal S22 improves slightly as the input power increases. Largesignal
S11 also improves at higher frequencies (5 and 8
GHz) as the input power increases. At low frequencies (2 GHz), however, S11 increases signi?cantly and leads to
rapid power gain compression.
Fig. 6(b) shows the large-signal transmission and
reˉection coe?cients of the ampli?er based on cascode design (design B). The large-signal forward transmission coe?cient S21 is found in this design to have a much
smaller dependence on input power. As the frequency increases, S21 decreases slightly for all shown input
power levels. The large-signal output reˉection coe?-
cient S22 is almost independent on input power for design
B at di.erent frequencies as is depicted in Fig. 6(b).
The large-signal input reˉection coe?cient S11, however, increases as the input power increases. This results in
power gain compression of the ampli?er at large input signal.
As mentioned earlier, in the case of the cascode transimpedance ampli?er of design B, the large signal S21 diminishes slightly as the input power is increased despite
the fact that self-biasing is not important (Fig.
6(b)). This is due to less e?cient ampli?cation in the frequency of interest under non-linear operation conditions where part of the injected carriers from the base to
the collector lead to higher harmonic frequency components. The latter is veri?ed by examining the output
power spectrum as the input signal is increased. This was done by performing harmonic-balanced simulation
based on the developed HBT large-signal model. As expected, the higher harmonic components of the output power of the ampli?er of designs A and B increase as the input incident power increases. Fig. 7 shows these features
by assuming that the fundamental frequency for
ampli?cation is 5 GHz and examining multiples of this frequency. One may observe that the output power lost
in harmonics is much higher in the case of design A at
power levels beyond ?12 dBm where the device behaves
in strongly non-linear fashion. As a result, the gain degradation with input power level is expected to be
smaller in design B.
The impact of input incident power on the power
gain of the transimpedance ampli?ers of designs A and
B was also investigated using the harmonic-balanced approach. Fig. 8(a) shows the power gain of design A
Fig. 6. Simulated Large-signal S-Parameters of (a) design A transimpedance ampli?er, (b) design B transimpedance ampli-
?er vs. input power at 2, 5 and 8 GHz frequency using HPEESOF LIBRA.
Fig. 7. Simulated fundamental and higher harmonic components
of output power as a function of input power for design A
and B at 5 GHz fundamental frequency.
2064 J.-W. Park et al. / Solid-State Electronics 44 (2000) 2059±2067 ampli?er as a function of frequency for di.erent input power. Notice that the power gain reduces dramatically
as the input power increases due to both self-biasing and
reduction of large-signal gain under non-linear operation conditions. Fig. 8(b) shows the power gain of design
B transimpedance ampli?er as a function of frequency
for di.erent input power levels. The reduction in gain is
less signi?cant due to the suppression of self-biasing.
The pronounced input mismatch as well as non-linear operation of the ampli?er are, however, found to reduce
the gain slightly at higher input power.
5. Experimental characteristics of the transimpedance
ampli?ers
The large signal performance of the two transimpedance designs was evaluated using an HP 8722D
network analyzer and the output power was monitored
with a power meter for higher accuracy. Fig. 9(a) and (b) shows the P out±P in characteristics for di.erent frequencies
for common-emitter based (design A) and cascode
based (design B) transimpedance ampli?ers, respectively. The chips compared showed similar gain-frequency characteristics under small-signal conditions. The common- emitter transimpedance ampli?er (design A) shows
a more pronounced gain compression especially at lower frequencies. The cascode transimpedance circuit, however, shows very small gain compression at high input
power. This is mainly due to the fact that self-biasing
e.ects resulting from the presence of large signal input conditions are reduced in this design as explained theoretically in Section 4.
Frequency dependence tests of the large-signal gain
proved again the superiority of the cascode design in
terms of immunity to increased input power level conditions. Fig. 8(a) and (b) shows a comparison of the
Fig. 8. Measured and harmonic-balanced simulation of power
gain vs. frequency as a function of input power for (a) design A transimpedance ampli?er, (b) design B transimpedance ampli-
?er.
Fig. 9. Measured P out±P in characteristics of (a) design A and (b) design B transimpedance ampli?ers.
J.-W. Park et al. / Solid-State Electronics 44 (2000) 2059±2067 2065 measured and simulated power gain vs. frequency characteristics for design A and B ampli?ers when the
input power is varied. The power gain of the cascode
based design (design B) shows a much smaller dependence on input power as the input power is varied over
the entire measured frequency range.
A comparison between the collector currents of
transistor T3 (output stage) of these two designs revealed
that in the case of design A, the collector current increases from the nominal value of 18±48 mA as the input
power increases from ?15 to ?3 dBm. The cascode
design however shows a negligible current increase.
Overall, the cascode design appears to be less sensitive to increased input power levels. This feature, in addition to
a higher bandwidth, make the cascode design a better choice for optoelectronic applications.
The eye diagrams of the two transimpedance ampli-
?ers were obtained using an Anritsu MP1701B 10 GHz random pattern generator and a Tektronix 11801B 50
GHz sampling oscilloscope. Both transimpedance ampli
?ers were subjected to a 215-1 NRZ PRBS signal at 10 GHz input data for eye diagram measurement. The resultant eye diagrams of designs A and B are shown in
Fig. 10(a) and (b), respectively. The cascode based design (design B) shows a much more open and clear eye
pattern even at smaller input excitation (Fig. 10(b)). The common-emitter based design (design A) showed an acceptable response at large input excitations (Fig.
10(a)). For smaller input signal, however, the eye pattern showed not only a more noisy amplitude of the output signal, but also non-consistent rise and fall times resulting
in a more closed and noisier eye pattern. This is
an indication of higher bit error rate at lower input excitation for transimpedance ampli?er of design A.
6. Conclusions
Broadband transimpedance ampli?ers were designed, fabricated and tested using an CBE-based GaInP/GaAs HBT technology which is promising due to the combination of good electrical performance with process
simplicity as necessary for production of high-speed and high capacity transmission systems. A maximum bandwidth of 19 GHz and associated transimpedance gain of
47 dBX were achieved using a cascode design approach. Two transimpedance designs were compared and the design based on the cascode approach showed larger bandwidth and a much smaller input power dependence. For the same gain characteristics, cascode con?guration requires a smaller collector resistance comparing to the common-emitter con?guration due to reduced Miller
e.ect in cascode design. This will translate into a smaller power supply requirement in cascode design for the
same optimum collector current. Therefore, the selfbiasing e.ect can be signi?cantly reduced in the cascode
con?guration resulting in a smaller sensitivity to input power. Eye diagrams of these two ampli?ers were measured at 10 GHz and a much more open and clear eye
pattern was achieved for the cascode con?guration. Acknowledgements
The authors would like to thank Dr. J.O. Plouchart
from IBM Watson Research Center for helpful discussions. This work is supported by CNET France- Telecom/DRI (contact no. 94 6M 917), Thomson-CSF,
US Army Research O?ce/ARO-URI (contact no.
DAAL03-92-G-0109).
Fig. 10. Output waveform eye diagram for 10 Gbs?1 215-1
NRZ input signal for (a) design A and (b) design B transimpedance ampli?ers.
2066 J.-W. Park et al. / Solid-State Electronics 44 (2000) 2059±2067 References
[1] Meyer RG, Mack WD. A wideband low-noise variablegain BiCMOS transimpedance ampli?er. IEEE J Solid-
State Circ 1994;29(6):701±6.
[2] Gutierrez-Aitken AL, Bhattacharya P, Chen YC, Pavlidis
D, Brock T. High-performance monolithic PIN-MODFET transimpedance photoreceiver. IEEE Photonics Technol
Lett 1993;5(8):913±5.
[3] Scheinberg N, Bayruns RJ, Laverick TM. Monolithic
GaAs transimpedance ampli?ers for ?ber-optic receivers.
IEEE J Solid-State Circ 1991;26(12):1834±9.
[4] van Waasen S, et al. 27-GHz bandwidth high-speed
monolithic integrated optoelectronic photoreceiver consisting
of a waveguide fed photodiode and an InAlAs/InGaAs-
HFET traveling wave ampli?er. IEEE J Solid-State Circ
1997;32(9):1394±401.
[5] Lunardi LM, Chandersekhar S, Gnauck AH, Burrus CA, Hamm RA, Sulho. JW, Zyskind JL. A 12 Gb/s highperformance, high-sensitivity monolithic pin/HBT photoreceiver
module for long-wavelength transmission systems.
IEEE Photonics Tech Lett 1995;7(2):182±4.
[6] Gutierrez-Aitken AL, Yang K, Zhang X, Haddad GI, Bhattacharya P, Lunardi LM. 16-GHz bandwidth InAlAs±
InGaAs monolithically integrated pin/HBT photoreceiver.
IEEE Photonics Tech Lett 1995;7(11):1339±41.
[7] Chandrasekhar S, Glance B, Dentai AG, Joyner CH, Qua
GJ, Sulho. JW. Monolithic balanced pin/HBT photoreceiver
for coherent optical heterodyne communications.
IEEE Photonic Tech Lett 1991;3(6):537±9.
[8] Suzuki Y, Shimawaki H, Amamiya Y, Fukuchi K. An
HBT preampli?er for 40 Gb/s optical systems. IEEE GaAs
IC Symp Dig 1996;203±6.
[9] Ihara T, Oikawa Y, Yamamoto T, Tomoguji H, Hamano
H, Ohnishi H, Watanabe Y. InGaP/GaAs HBT IC chipset
for 10 Gb/s optical receiver. IEEE GaAs IC Symp Dig
1996;262±5.
[10] Takahashi T, Sasa S, Kawano A, Iwai T, Fujii T. Highreliability InGaP/GaAs HBTs fabricated by self-aligned
process. 1994 IEDM Tech Dig 1994;191.
[11] Razeghi M, Omnes F, Defour M, Maurel Ph, Hu J,
Pavlidis D. High performance GaAs/GaInP HBTs grown
by MOCVD. Sem Sci Tech 1990;5:278±80.
[12] Fresina MT, Ahmari DA, Mares PJ, Hartmann QJ, Feng
M, Stillman GE. High-speed, low-noise InGaP/GaAs
HBTs. IEEE Electron Dev Lett 1995;16(12):540±1.
[13] Park JW, Mohammadi S, Pavlidis D, Dua C, Garcia J-C.
GaInP/GaAs HBT Broadband Monolithic Transimpedance
Ampli?ers and their High-Frequency small and large
signal characteristics. IEEE MTTS International Microwave Symposium Technical Digest, (IEEE Radio Frequency
Integrated Circuits, RFIC ?98). Baltimore MD,
1998;1: 39±42.
[14] Nagano N, Suzaki T, Kasahara K, Takeuchi T, Honjo K. Monolithic ultra-broadband transimpedance ampli?ers
using AlGaAs/GaAs HBTs. IEEE Trans Microwave Theory
Tech 1994;42(1):2±9.
[15] Park JW, Pavlidis D, Mohammadi S, Guyaux J-L,
Garcia J-C. High speed tunneling emitter GaInP/GaAs
HBTs. IEEE Trans Electron Dev 2000, submitted for publication. [16] Garcia JC, Dua C, Mohammadi S, Park JW, Pavlidis D.
Growth characterization of Hydride-free CBE and application
of GaInP/GaAs HBTs. J Electron Mater 1998;
27(5):442±5.
[17] Pehlke D, Pavlidis D. Evaluation of the factors determining HBT high-frequency performance by direct analysis of
S-parameter data. IEEE Trans Microwave Theory Tech
1992;40:2367±73.
[18] Samelis A, Pavlidis D. Analysis of the large-signal characteristics of power HBTs exhibiting self-heating e.ects.
IEEE Trans Microwave Theory Tech 1997;45:534±42.
J.-W. Park et al. / Solid-State Electronics 44 (2000) 2059±2067 2067
电线、电流、功率之间的换算
1、16平方线承载多大电流80A 2、16平方线铜线承载多大电流100A 380V每KW2A 660V每 3000V4KW1A 6000V电动机2KW1A 导线截面积与的一般计算 一、一般铜导线的安全是根据所允许的线芯最高温度、冷却条件、敷设条件来确定的。一般铜导线的安全为5~8A/mm2,铝导线的安全载流量为3~5A/mm2。<关键点> 一般铜导线的安全载流量为5~8A/mm2,铝导线的安全载流量为3~5A/mm2。如:mm2 BVV铜导线安全载流量的推荐值×8A/mm2=20A 4 mm2 BVV铜导线安全载流量的推荐值4×8A/mm2=32A 二、计算铜导线截面积利用铜导线的安全载流量的推荐值5~8A/mm2,计算出所选取铜导线截面积S的上下范围:S=< I /(5~8)>= I ~ I(mm2)S-----铜导线截面积(mm2)I-----(A)三、算一般负载(也可以成为用电器,如点灯、冰箱等等)分为两种,一种式电,一种是电。对于电的计算公式:P=UI 对于负载的计算公式:P=UIcosф,其中负载的cosф=。不同电不同,统一计算家庭用电器时可以将cosф取。也就是说如果一个家庭所有用电器加上总功率为6000瓦,则最大电流是I=P/Ucosф=6000/220*=34(A) 但是,一般情况下,家里的电器不可能同时使用,所以加上一个公用系数,公用系数一般。所以,上面的计算应该改写成I=P*数/Ucosф=6000*220*=17(A) 也就是说,这个家庭总的电流值为17A。则总闸不能使用16A,应该用大于17A的。 估算口诀: 二点五下乘以九,往上减一顺号走。 三十五乘三点五,双双成组减点五。 条件有变加折算,高温九折铜升级。 穿管根数二三四,八七六折满载流。 说明:
常用热量单位换算表
常用热量单位换算表 1KJ=1000J 1MJ=1000KJ=1000000J 1GJ=1000MJ=1000000KJ=00J 1焦耳= 因此1千瓦时=1000W×3600秒=3600千焦 供热热量单位换算与节能计算实例 单位换算与计算虽然论坛经常讨论,在节能减排严峻形势下,大家关注程度更加高涨,真是可喜可贺啊!最近,我编制了几篇公司供热节能指标考核文件,将大家比较关心的主要问题与大家交流一下,以望共同提高: % 1、能量、能耗、热耗、热量等一系列术语在供热领域其含义及单位是一致的,大家不必要去怎样表达,这些物理量在结合时间、空间等条件时在计算上就变得复杂起来,所以,供热一般计算时一定理解物理量含义,而不必要理会推导过程,去除有些条件,使计算变得简化。 2、热量单位常用的三种形式,大家要分清哪种单位是常用的及应用表达环境,分述如下: (1)、焦耳(J)、千焦(KJ)、吉焦(GJ),工程计算广为采用,国际单位制。热力计算、热计量、热量化验等实际操作中常见,国家标准及图表、线图查询等规范性技术文件中主要表达的单位。但是,其他导出单位及工程习惯相互交织,使得这种单位在今天热力计算中不是很方便。 (2)、瓦特(W)、千瓦(KW)、兆瓦(MW),工程导出单位,是供热工程常用单位,如热水锅炉热容量:7MW、14MW、29MW、56MW...等,习惯上常说到的10t、20t、40t、80t...等锅炉,相当于同类容量蒸汽锅炉的设计出力.工程上热水锅炉和换热站热计量仪表、暖通供热设计计算、估算、供热指标等,广泛采用。
(3)、卡(car)、千卡(Kcal)...,已经淘汰的热量单位,但是工程中还在使用,特别是大量的技术书籍,例如煤的标准发热量7000KCal,等. 3、供热指标核算、计算及测算时,我给大家推荐4个基本换算式,在这些工作计算的结果,虽然有点误差,但是已足够精确。如果一定精确计算,则要查有关图表手册了: (1)、1W=, 1Kcal=; (2)、1t饱和蒸汽===60万Kcal; (3)、1kg标煤=7000Kcal=29300KJ; (4)、热工当量1Kcal=. 1W=(热工当量是换算式,不是物理关系式,热力计算常用). 4、北京地区供热实现节能的主要指标值(采暖期4个月) # (1)、第一步节能:建筑热耗m2,煤耗标煤/m2; (2)、第二步节能:建筑热耗m2,煤耗标煤/m2; (3)、第三步节能:建筑热耗m2,煤耗标煤/m2. 注:北京第一步节能主要指标为2000年前最好水平,各地参照时,先将此4个月的指标值折算一个月,北方地区采暖期有6各月或7各月的,按采暖期制定考核指标,月考核先挂账,终了节能绩效结算. 5、能耗指标考核计算实例 (1)、例1,我热力公司呼和浩特某开发区8座汽水换热站2010年制定节能指标考核管理办法,当地供暖期为6个月,统计连续3年采暖期的能耗年报表,经过数据分析及价格测算,初步确定了各换热站汽耗指标为160Kg汽/m2,整个采暖期.已知,当地设计面积热指标是65W,各站蒸汽计量,蒸汽为饱和蒸汽,工作压力小于.问采暖期折算每平米标煤是多少采暖期每平米折算热量是多少与北京地区哪个节能指标较接近 解:A,×=112000W=130256Kcal; B,130256/7000=标煤,(接近北京第一步节能指标,按6个月折算,×6/4=标煤); C,112000w/180d/24h=m2.(远低于设计值) 关于确定煤耗指标先从技术角度分析,同时必须考虑燃料的价格,因为节能指标的制定,是从技术经济角度找出能源管理的盈亏点,方可真正找出节能的途径,不可玩起数字游戏.定汽耗指标时,要测算蒸汽生产成本及管损才有意义. ~ (2)、例2,我公司另一座热水锅炉房,没有上供热热水计量仪表,制定出6个月采暖期煤耗指标为:43Kg煤/m2,煤的低位发热量为5400Kcal,问折算成采暖期每平米汽耗是多少采暖期每平米热耗是多少折算标煤是多少 解:a,43×5400=232200Kcal=270049W; b,270049/700000=(饱和蒸汽); c,270049w/180d/24h=m2,6个月; d,折算标煤:43×5400/7000=.(如果采暖期每平米按标煤订煤耗指标,就是这个计算值,也不一定有实际意义). 这个实例提供了热计量及燃料计量.电计量、水计量等在考核范围内一切物化、量化方面计量的重要意义,它可以通过计量统计,加强节能考核及分析,
功率电流快速计算公式
功率电流快速计算公式2012-6-10 功率电流快速计算公式,导线截面积与电流的关系 功率电流速算公式: 三相电机: 2A/KW 三相电热设备:1.5A/KW 单相220V, 4.5A/KW 单相380V, 2.5A/KW 铜线、铝线截面积(mm2)型号系列: 1 1.5 2.5 4 6 10 16 25 35 50 70 95 120 150 185 .......一般铜线安全电流最大为: 2.5平方毫米铜电源线的安全载流量--28A。 4平方毫米铜电源线的安全载流量--35A 。 6平方毫米铜电源线的安全载流量--48A 。 10平方毫米铜电源线的安全载流量--65A。 16平方毫米铜电源线的安全载流量--91A 。 25平方毫米铜电源线的安全载流量--120A。
如果是铝线截面积要取铜线的1.5-2倍。 如果铜线电流小于28A,按每平方毫米10A来取肯定安全。 如果铜线电流大于120A,按每平方毫米5A来取。 铝导线的截面积所能正常通过的电流可根据其所需要导通的电流总数进行选择,一般可按照如下顺口溜进行确定: 十下五,百上二, 二五三五四三倍,七零九五两倍半,铜线升级算. 就是10平方以下的铝线,平方毫米数乘以5就可以了,要是铜线呢,就升一个档,比如2.5平方的铜线,就按铝线4平方计算.一百以上的都是截面积乘以2, 二十五平方以下的乘以4, 三十五平方以上的乘以3, 70和95平方都乘以2.5。 说明:只能作为估算,不是很准确。 另外如果是室内,6平方毫米以下的铜线,每平方电流不超过10A就是安全的,从这个角度讲,可以选择1.5平方的铜线或2.5平方的铝线。 10米内,导线电流密度6A/平方毫米比较合适,10-50米,3A/平方毫米,50-200米,2A/平方毫米,500米以上要小于1A/平方毫米。从这个角度,如果不是很远的情况下,可以选择4平方铜线或者6平方铝线。 如果是距离150米供电,一定采用4平方的铜线。 导线的阻抗与其长度成正比,与其线径成反比。在使用电源时,特别要注意输入与输出导线的线材与线径问题。以防止电流过大使导线过热而造成事故。
常用热力单位换算表
常用热力单位换算表 一、热量单位换算 1、常用热量单位介绍 A、焦耳(J)、千焦(KJ)、吉焦(GJ),工程计算广为采用,国际单位制。热力计算、热计量、热量化验等实际操作中常见,国家标准及图表、线图查询等规范性技术文件中主要表达的单位。但是,其他导出单位及工程习惯相互交织,使得这种单位在今天热力计算中不是很方便。 B、瓦特(W)、千瓦(KW)、兆瓦(MW),工程导出单位,是供热工程常用单位,如热水锅炉热容量:7MW、14MW、29MW、56MW...等,习惯上常说到的10t、20t、40t、80t...等锅炉,相当于同类容量蒸汽锅炉的设计出力.工程上热水锅炉和换热站热计量仪表、暖通供热设计计算、估算、供热指标等,广泛采用。 C、卡(car)、千卡(Kcal)...,已经淘汰的热量单位,但是工程中还在使用,特别是大量的技术书籍,例如煤的标准发热量7000Kcal。 2、基本计算公式 1W=0.86Kcal,1KW=860Kcal,1Kcal=1.163W; 1t饱和蒸汽=0.7MW=700KW=2.5GJ=60万Kcal; 1kg标煤=7000Kcal=29300KJ=29.3MJ=0.0293GJ=8141W=8.141KW; 1GJ=1000MJ;1MJ=1000KJ;1KJ=1000J 1Kcal=4.1868KJ 1W=3.6J(热工当量,不是物理关系,但热力计算常用)
4、制冷机热量换算 1美国冷吨=3024千卡/小时(kcal/h)=3.517千瓦(KW) 1日本冷吨=3320千卡/小时(kcal/h)=3.861千瓦(KW) 1冷吨就是使1吨0℃的水在24小时内变为0℃的冰所需要的制冷量。) 1马力(或1匹马功率)=735.5瓦(W)=0.7355千瓦(KW) 1千卡/小时(kcal/h)=1.163瓦(W) 二、压力单位换算 1、1Mpa=1000Kpa;1Kpa=1000pa 2、1标准大气压=0.1Mp=1标准大气压 1标准大气压=1公斤压力=100Kpa=1bar 1mmHg = 13.6mmH20 = 133.32 Pa(帕) 1mmH20=10Pa(帕) 1KPa=1000Pa=100mmH20(毫米水柱) 1bar=1000mbar 1mbar=0.1kpa=100pa
几种常用单位换算表
几种常用法定计量单位换算表 我国的法定计量单位(以下简称法定单位)包括: 1.国际单位制的基本单位; 2.国际单位制的辅助单位; 3.国际单位制中具有专门名称的导出单位; 4.国家选定的非国际单位制单位; 5.由以上单位构成的组合形式的单位; 6.由词头和以上单位所构成的十进倍数和分数单位。 国际单位制中具有专门名称的导出单位 量的名称单位名称单位符号其它表示式例频率赫[兹] Hz s-1 力、重力牛[顿] N kg?m/s2 压力、压强、应力帕[斯卡] Pa N/m2 能量、功、热焦[耳] J N?m 功率、辐射通量瓦[特] W J/s 电荷量库[仑] C A?s 电位、电压、电动势伏[特] V W/A 电容法[拉] F C/V 电阻欧[姆] S V/A 电导西[门子] Wb A/V 磁通量韦[伯] T V?s 磁通量密度、磁感应强度特[斯拉] H Wb/m2 电感亨[利] C Wb/A 摄氏温度摄氏度1m cd?sr 光通量流[明] 1x 1m/ m2 光照度勒[克斯] Bq s-1 放射性活度贝可[勒尔] Gy J/kg 吸收剂量戈[瑞] Sv J/kg 剂量当量希[沃特] 国家选定的非国际单位制单位 量的名称单位名称单位符号换算关系和说明 时间分 [小]时 天(日) min h d 1min=60s 1h=60min=3600s 1d=24h=86400s 平面角[角]秒 [角]分 度 (″) (′) (°) 1″=( π/640800)rad (π为圆周率) 1′=60″=(π/10800)rad
1°=60′=(π/180)rad 旋转速度转每分r/min 1r/min=(1/60)s-1 长度海里n mile 1n mile=1852m (只用于航行) 速度节kn 1kn=1n mile/h =(1852/3600)m/s (只用于航行) 质量吨原子质量单位t u 1t=103kg 1u≈1.6605655×10-27kg 体积升L,(1) 1L=1dm3=10-3m3 能电子伏eV 1eV≈1.6021892×10-19J 级差分贝dB 线密度特[克斯] tex 1tex=1g/km 常用压力单位换算表 https://www.360docs.net/doc/fa2205748.html,/valves/units-conversion.gif
功率电压电流公式 功率电压电流公式大全
功率电压电流公式功率电压电流公式大全 1、欧姆定律: I=U/R U:电压,V; R:电阻,Ω; I:电流,A; 2、全电路欧姆定律: I=E/(R+r) I:电流,A; E:电源电动势,V; r:电源内阻,Ω; R:负载电阻,Ω 3、并联电路,总电流等于各个电阻上电流之和 I=I1+I2+…In 4、串联电路,总电流与各电流相等 I=I1=I2=I3=…=In 5、负载的功率 纯电阻有功功率P=UI → P=I2R(式中2为平方) U:电压,V; I:电流,A; P:有功功率,W; R:电阻
纯电感无功功率Q=I2*Xl(式中2为平方)Q:无功功率,w; Xl:电感感抗,Ω I:电流,A 纯电容无功功率Q=I2*Xc(式中2为平方)Q:无功功率,V; Xc:电容容抗,Ω I:电流,A 6、电功(电能) W=UIt W:电功,j; U:电压,V; I:电流,A; t:时间,s 7、交流电路瞬时值与最大值的关系 I=Imax×sin(ωt+Φ) I:电流,A; Imax:最大电流,A; (ωt+Φ):相位,其中Φ为初相。 8、交流电路最大值与在效值的关系 Imax=2的开平方×I I:电流,A; Imax:最大电流,A; 9、发电机绕组三角形联接
I线=3的开平方×I相 I线:线电流,A; I相:相电流,A; 10、发电机绕组的星形联接 I线=I相 I线:线电流,A; I相:相电流,A; 11、交流电的总功率 P=3的开平方×U线×I线×cosΦ P:总功率,w; U线:线电压,V; I线:线电流,A; Φ:初相角 12、变压器工作原理 U1/U2=N1/N2=I2/I1 U1、U2:一次、二次电压,V; N1、N2:一次、二次线圈圈数; I2、I1:二次、一次电流,A; 13、电阻、电感串联电路 I=U/Z Z=(R2+XL2)和的开平方(式中2为平方) Z:总阻抗,Ω; I:电流,A; R:电阻,Ω; XL:感抗,Ω 14、电阻、电感、电容串联电路 I=U/Z Z=[R2+(XL-Xc)2]和的开平方(式中2为平方)Z:总阻抗,Ω; I:电流,A; R:电阻,Ω; XL:感抗,Ω; Xc:容抗,Ω
常用法定计量单位换算表
常用法定计量单位换算表 我国的法定计量单位(以下简称法定单位)包括: 1.国际单位制的基本单位; 2.国际单位制的辅助单位; 3.国际单位制中具有专门名称的导出单位; 4.国家选定的非国际单位制单位; 5.由以上单位构成的组合形式的单位; 6.由词头和以上单位所构成的十进倍数和分数单位。 国际单位制中具有专门名称的导出单位 量的名称单位名称单位符号其它表示式例频率赫[兹] Hz s-1 力、重力牛[顿] N kg?m/s2 压力、压强、应力帕[斯卡] Pa N/m2 能量、功、热焦[耳] J N?m 功率、辐射通量瓦[特] W J/s 电荷量库[仑] C A?s 电位、电压、电动势伏[特] V W/A 电容法[拉] F C/V 电阻欧[姆] S V/A 电导西[门子] Wb A/V 磁通量韦[伯] T V?s 磁通量密度、磁感应强度特[斯拉] H Wb/m2 电感亨[利] C Wb/A 摄氏温度摄氏度1m cd?sr 光通量流[明] 1x 1m/ m2
光照度勒[克斯] Bq s-1 放射性活度贝可[勒尔] Gy J/kg 吸收剂量戈[瑞] Sv J/kg 剂量当量希[沃特] 国家选定的非国际单位制单位 量的名称单位名称单位符号换算关系和说明 时间分 [小]时 天(日) min h d 1min=60s 1h=60min=3600s 1d=24h=86400s 平面角[角]秒 [角]分 度 (″) (′) (°) 1″=( π/640800)rad (π为圆周率) 1′=60″=(π/10800)rad 1°=60′=(π/180)rad 旋转速度转每分r/min 1r/min=(1/60)s-1 长度海里n mile 1n mile=1852m (只用于航行) 速度节kn 1kn=1n mile/h =(1852/3600)m/s (只用于航行) 质量吨原子质量单位t u 1t=103kg 1u≈1.6605655×10-27kg 体积升L,(1) 1L=1dm3=10-3m3 能电子伏eV 1eV≈1.6021892×10-19J 级差分贝dB 线密度特[克斯] tex 1tex=1g/km 压力等级Class和公称压力对照表
电流、电压、功率的关系及公式
电流=电压/电阻 功率=电压*电流*时间 电流I,电压V,电阻R,功率W,频率F W=I的平方乘以R V=IR 电流I,电压V,电阻R,功率W,频率F W=I的平方乘以R V=IR W=V的平方除以R 电压V(伏特),电阻R(欧姆),电流强度I(安培),功率N(瓦特)之间的关系是: V=IR,N=IV =I*I*R, 或也可变形为:I=V/R,I=N/V等等.但是必须注意,以上均是在直流(更准确的说,是直流稳态)电路情况下推导出来的!其它情况不适用.如交流电路,那要对其作补充和修正求电压、电阻、电流与功率的换算关系 电流=I,电压=U,电阻=R,功率=P U=IR,I=U/R,R=U/I, P=UI,I=P/U,U=P/I P=U2/R,R=U2/P 就记得这一些了,不知还有没有 还有P=I2R P=IU R=U/I 最好用这两个;如电动机电能转化为热能和机械能。电流 符号: I 符号名称: 安培(安) 单位: A 公式: 电流=电压/电阻 I=U/R 单位换算: 1MA(兆安)=1000kA(千安)=1000000A(安) 1A(安)=1000mA(毫安)=1000000μA(微安)单相电阻类电功率的计算公式= 电压U*电流I
单相电机类电功率的计算公式= 电压U*电流I*功率因数COSΦ 三相电阻类电功率的计算公式= *线电压U*线电流I (星形接法) = 3*相电压U*相电流I(角形接法) 三相电机类电功率的计算公式= *线电压U*线电流I*功率因数COSΦ(星形电流=I,电压=U,电阻=R,功率=P U=IR,I=U/R,R=U/I, P=UI,I=P/U,U=P/I P=U2/R,R=U2/P 就记得这一些了,不知还有没有 还有P=I2R ⑴串联电路 P(电功率)U(电压)I(电流)W(电功)R(电阻)T(时间) 电流处处相等 I1=I2=I 总电压等于各用电器两端电压之和 U=U1+U2 总电阻等于各电阻之和 R=R1+R2 U1:U2=R1:R2 总电功等于各电功之和 W=W1+W2 W1:W2=R1:R2=U1:U2 P1:P2=R1:R2=U1:U2 总功率等于各功率之和 P=P1+P2 ⑵并联电路 总电流等于各处电流之和 I=I1+I2 各处电压相等 U1=U1=U 总电阻等于各电阻之积除以各电阻之和R=R1R2÷(R1+R2) 总电功等于各电功之和 W=W1+W2 I1:I2=R2:R1 W1:W2=I1:I2=R2:R1 P1:P2=R2:R1=I1:I2 总功率等于各功率之和 P=P1+P2
功率换算电流简单算法
功率换算电流简单算法 单相220V: (照明等)每千瓦等于4.5 A 在380/220伏三相四线系统中,单相设备的两条线,一条接相线而另一条接零线的(如照明设备)为单相220伏用电设备。这种设备的力率大多为1,因此,便直接说明“单相(每)千瓦4.5安”。计算时,只要“将千瓦数乘4.5”就是电流,安。同上面一样,它适用于所有以千伏安为单位的单相220伏用电设备,以及以千瓦为单位的电热及照明设备,而且也适用于220伏的直流 三相380V: 电力(电动机等)每千瓦约为 2 A 电力专指电动机.在380V三相时(力率0.8左右),电动机每千瓦的电流约为2安.即将“千瓦数加一倍”(乘2)就是电流,安。这电流也称电动机的额定电流. 电热(热水器等)每千瓦等于 1.5A 三相380伏的电热设备,每千瓦的电流为1.5安。即将“千瓦数加一半”(乘1.5),就是电流,安 电流(A)=功率(W)÷(1.732×(380V或220V)电压×0.8(功率因数)) 1000W÷(1.732×380V×0.8)=1.89A 1000W÷(1.732×220V×0.8)=3.28A 功率(W)=电压(V)×电流(A)×时间(H) 220V×3.28A×1H=721W 380V×1.89A×1H=718.2W 《电流换算电线》 二点五下乘以九,往上减一顺号走。 三十五乘三点五,双双成组减点五。 条件有变加折算,高温九折铜升级。 穿管根数二三四,八七六折满载流。 二点五下乘以九,往上减一顺号走。 "说的是2.5mm’及以下的各种截面铝芯绝缘线,其载流量约为截面数的9倍。如2.5mm’导线,载流量为2.5×9=22.5(A)。从4mm’及以上导线的载流量和截面数的倍数关系是顺着线号往上排,倍数逐次减l,即4×8、6×7、10×6、16×5、25×4 三十五乘三点五,双双成组减点五。 说的是35mm"的导线载流量为截面数的3.5倍,即35×3.5=122.5(A)。从50mm’及以上的导线,其载流量与截面数之间的倍数关系变为两个两个线号成一组,倍数依次减0.5。即50、70mm’导线的载流量为截面数的3倍;95、120mm"导线载流量是其截面积数的2.5
电线、电流、功率之间的换算
1、16平方线铝线承载多大电流80A 2、16平方线铜线承载多大电流100A 380V电动机每KW2A 660V电动机每KW1.2A 3000V电动机4KW1A 6000V电动机2KW1A 导线截面积与载流量的一般计算 一、一般铜导线载流量导线的安全载流量是根据所允许的线芯最高温度、冷却条件、敷设条件来确定的。一般铜导线的安全载流量为5~8A/mm2,铝导线的安全载流量为3~5A/mm2。<关键点> 一般铜导线的安全载流量为5~8A/mm2,铝导线的安全载流量为3~5A/mm2。如:2.5 mm2 BVV铜导线安全载流量的推荐值2.5×8A/mm2=20A 4 mm2 BVV铜导线安全载流量的推荐值4×8A/mm2=32A 二、计算铜导线截面积利用铜导线的安全载流量的推荐值5~8A/mm2,计算出所选取铜导线截面积S的上下范围:S=< I /(5~8)>=0.125 I ~0.2 I(mm2)S-----铜导线截面积(mm2)I-----负载电流(A) 三、功率计算一般负载(也可以成为用电器,如点灯、冰箱等等)分为两种,一种式电阻性负载,一种是电感性负载。对于电阻性负载的计算公式:P=UI 对于日光灯负载的计算公式:P=UIcosф,其中日光灯负载的功率因数cosф=0.5。不同电感性负载功率因数不同,统一计算家庭用电器时可以将功率因数cosф取0.8。也就是说如果一个家庭所有用电器加上总功率为6000瓦,则最大电流是I=P/Ucosф=6000/220*0.8=34(A) 但是,一般情况下,家里的电器不可能同时使用,所以加上一个公用系数,公用系数一般0.5。所以,上面的计算应该改写成I=P*数/Ucosф=6000*0.5/220*0.8=17(A) 也就是说,这个家庭总的电流值为17A。则总闸空气开关不能使用16A,应该用大于17A的。 估算口诀: 二点五下乘以九,往上减一顺号走。 三十五乘三点五,双双成组减点五。 条件有变加折算,高温九折铜升级。 穿管根数二三四,八七六折满载流。 说明: (1)本节口诀对各种绝缘线(橡皮和塑料绝缘线)的载流量(安全电流)不是直接指出,而是“截面乘上一定的倍数”来表示,通过心算而得。由表5 3可以看出:倍数随截面的增大而减小。“二点五下乘以九,往上减一顺号走”说的是2.5mm’及以下的各种截面铝芯绝缘线,其载流量约为截面数的9倍。如2.5mm’导线,载流量为2.5×9=22.5(A)。从4mm’及以上导线的载流量和截面数的倍数关系是顺着线号往上排,倍数逐次减l,即4×8、6×7、10×6、16×5、25×4。 “三十五乘三点五,双双成组减点五”,说的是35mm”的导线载流量为截面数的3.5倍,即
功率电流快速计算公式
功率电流快速计算公式 Revised as of 23 November 2020
功率电流快速计算公式,导线截面积与电流的关系 功率电流速算公式: 三相电机: 2A/KW 三相电热设备:1.5A/KW 单相220V, 4.5A/KW 单相380V, 2.5A/KW 铜线、铝线截面积(mm2)型号系列: 1 4 6 10 16 25 35 50 70 95 120 150 185 ....... 一般铜线安全电流最大为: 平方毫米铜电源线的安全载流量--28A。 4平方毫米铜电源线的安全载流量--35A 。 6平方毫米铜电源线的安全载流量--48A 。 10平方毫米铜电源线的安全载流量--65A。 16平方毫米铜电源线的安全载流量--91A 。 25平方毫米铜电源线的安全载流量--120A。 如果是铝线截面积要取铜线的倍。 如果铜线电流小于28A,按每平方毫米10A来取肯定安全。 如果铜线电流大于120A,按每平方毫米5A来取。 铝导线的截面积所能正常通过的电流可根据其所需要导通的电流总数进行选择,一般可按照如下顺口溜进行确定: 十下五,百上二, 二五三五四三倍,七零九五两倍半,铜线升级算.
就是10平方以下的铝线,平方毫米数乘以5就可以了,要是铜线呢,就升一个档,比如平方的铜线,就按铝线4平方计算.一百以上的都是截面积乘以2, 二十五平方以下的乘以4, 三十五平方以上的乘以3, 70和95平方都乘以。 说明:只能作为估算,不是很准确。 另外如果是室内,6平方毫米以下的铜线,每平方电流不超过10A就是安全的,从这个角度讲,可以选择平方的铜线或平方的铝线。 10米内,导线电流密度6A/平方毫米比较合适,10-50米,3A/平方毫米,50-200米,2A/平方毫米,500米以上要小于1A/平方毫米。从这个角度,如果不是很远的情况下,可以选择4平方铜线或者6平方铝线。 如果是距离150米供电,一定采用4平方的铜线。 导线的阻抗与其长度成正比,与其线径成反比。在使用电源时,特别要注意输入与输出导线的线材与线径问题。以防止电流过大使导线过热而造成事故。 下面是铜线在不同温度下的线径和所能承受的最大电流:
功率算电流计算口诀
功率算电流|电源功率计算方法|线缆电流计算 一、按功率计算电流的口诀之一 1、用途 这是根据用电设备的功率(千瓦或千伏安)算出电流(安)的口诀。 电流的大小直接与功率有关,也与电压、相别、力率(又称功率因数)等有关。一般有公式可供计算。由于工厂常用的都是380/220伏三相四线系统,因此,可以根据功率的大小直接算出电流。 2、口诀 低压380/220伏系统每千瓦的电流,安。 千瓦、电流,如何计算?电力加倍,电热加半。①单相千瓦,4.5安。 ②单相380,电流两安半。③ 3、说明 口诀是以380/220伏三相四线系统中的三相设备为准,计算每千瓦的安数。对于某些单相或电压不同的单相设备,其每千瓦的安数,口诀另外作了说明。 ①这两句口诀中,电力专指电动机。在380伏三相时(力率0.8左右),电动机每千瓦的电流约为2安。即将“千瓦数加一倍”(乘2)就是电流,安。这电流也称电动机的额定电流。 [例1] 5.5千瓦电动机按“电力加倍”算得电流为11安。① [例2] 40千瓦水泵电动机按“电力加倍”算得电流为80安。 电热是指用电阻加热的电阻炉等。三相380伏的电热设备,每千瓦的电
流为1.5安。即将“千瓦数加一半”(乘1.5)就是电流,安。 [例1] 3千瓦电加热器“电热加半”算得电流为4.5安。 [例2] 15千瓦电阻炉按“电热加半”算得电流为23安。② 这口诀并不专指电热,对于照明也适用。虽然照明的灯泡是单相而不是三相,但对照明供电的三相四线干线仍属三相。只要三相大体平衡也可这样计算。此外,以千伏为单位的电器(如变压器或整流器)和以千乏为单位的移相电容器(提高力率用)也都适用。即是说,这后半句虽然说的是电热,但包括所有以千伏安、千乏为单位的用电设备,以及以千瓦为单位的电热和照明设备。 [例1] 12千瓦的三相(平衡时)照明干线按“电热加半”算得电流为18安。 [例2] 30千伏安的整流器按“电热加半”算得电流为45安(指380伏三相交流侧)。 备注:①按“电力加倍”计算电流,与电动机铭牌上的电流有的有些误差。一般千瓦数较大的,算得的电流比铭牌上的略大些,而千瓦数较小的,算得的电流则比铭牌上的略小些。此外,还有一些影响电流大小的因素。不过,作为估算,影响并不大。 ②计算电流时,当电流达到十多按或几十安以上,则不必算到小数点以后,可以四舍五入成整数。这样既简单又不影响实用。对于较小的电流也只要算到一位小数即可。 [例3] 320千伏安的配电变压器按“电热加半”算得电流为480安(指 380/220伏低压侧)。
电机转矩、功率、转速、电压、电流之间的关系及计算公式
电机转矩、功率、转速之间的关系及计算公式 电动机输出转矩: 使机械元件转动的力矩称为转动力矩,简称转矩。机械元件在转矩作用下都会产生 一定程度的扭转变形,故转矩有时又称为扭矩。 转矩与功率及转速的关系:转矩(T)=9550*功率(P)/转速(n) 即:T=9550P/n—公式【1】 由此可推导出: 转矩=9550*功率/转速《===》功率=转速*转矩/9550,即P=Tn/9550——公式【2】 方程式中: P—功率的单位(kW); n—转速的单位(r/min); T—转矩的单位(N.m); 9550是计算系数。 电机扭矩计算公式T=9550P/n 是如何计算的呢? 分析: 功率=力*速度即P=F*V---————公式【3】转矩(T)=扭力(F)*作用半径(R) 推出F=T/R---——公式【4】 线速度(V)=2πR*每秒转速(n秒)=2πR*每分转速(n分)/60=πR*n分/30---——公式【5】 将公式【4】、【5】代入公式【3】得: P=F*V=T/R*πR*n分/30 =π/30*T*n分 -----P=功率单位W, T=转矩单位N.m, n分=每分钟转速单位转/分钟 如果将P的单位换成KW,那么就是如下公式: P*1000=π/30*T*n 30000/π*P=T*n 30000/3.1415926*P=T*n 9549.297*P=T*n 这就是为什么会有功率和转矩*转速之间有个9550的系数关系。。。 电动机转矩、转速、电压、电流之间的关系 由于电功率P=电压U*电流I,即 P=UI————公式【6】 由于公式【2】中的功率P的单位为kw,而电压U的单位是V,电流I的单位是A,而UI乘积的单位是V.A,即w,所以将公式【6】代入到公式【2】中时,UI需要除以1000以统一单位。 则: P=Tn/9550=UI/1000————公式【7】
电流功率换算
功率电流速算公式: 三相电机:2A/KW 三相电热设备:1.5A/KW 单相220V, 4.5A/KW 单相380V, 2.5A/KW 铜线、铝线截面积(mm2)型号系列: 1 1.5 2.5 4 6 10 16 25 35 50 70 95 120 150 185 ....... 一般铜线安全电流最大为: 2.5平方毫米铜电源线的安全载流量--28A。 4平方毫米铜电源线的安全载流量--35A 。 6平方毫米铜电源线的安全载流量--48A 。 10平方毫米铜电源线的安全载流量--65A。 16平方毫米铜电源线的安全载流量--91A 。 25平方毫米铜电源线的安全载流量--120A。 如果是铝线截面积要取铜线的1.5-2倍。 如果铜线电流小于28A,按每平方毫米10A来取肯定安全。 如果铜线电流大于120A,按每平方毫米5A来取。 铝导线的截面积所能正常通过的电流可根据其所需要导通的电流总数进行选择,一般可按照如下顺口溜进行确定: 十下五,百上二, 二五三五四三倍,七零九五两倍半,铜线升级算. 就是10平方以下的铝线,平方毫米数乘以5就可以了,要是铜线呢,就升一个档, 比如2.5平方的铜线,就按铝线4平方计算.一百以上的都是截面积乘以2, 二十五平方以下的乘以4, 三十五平方以上的乘以3, 70和95平方都乘以2.5。 说明:只能作为估算,不是很准确。 另外如果是室内,6平方毫米以下的铜线,每平方电流不超过10A就是安全的,从这个角度讲,可以选择1.5平方的铜线或2.5平方的铝线。 10米内,导线电流密度6A/平方毫米比较合适,10-50米,3A/平方毫米,50-200米,2A/平方毫米,500米以上要小于1A/平方毫米。从这个角度,如果不是很远的情况下,可以选择4平方铜线或者6平方铝线。
醇基燃料能量换算表
醇基燃料能量换算表公司标准化编码 [QQX96QT-XQQB89Q8-NQQJ6Q8-MQM9N]
1.常用单位 2.常用单位换算 3.常用公式 锅炉等功率换算: 1MW=1000KW =1T 1T=60万大卡/小时1万大卡/小时= =3T锅炉
#:1公斤醇基燃料能够产生的蒸汽 按照200度的饱和蒸汽,压力为,焓值为 KJ/Kg,20度给水的焓值为84 KJ/Kg,为80%来算的话 5500kcal/kg×= KJ/Kg 所以每公斤醇基燃料产生蒸汽为*()=蒸汽 一.经济性比较 这里以4吨蒸汽锅炉为例(1吨蒸汽需要60W大卡),见表2-1
表 2-14吨蒸汽锅炉不同燃料经济对比由以上对比可以看出,工业醇基燃料油比柴油节省8%左右,比天然气高出约12%左右,但是天然气需要额外支付昂贵的管道开口费,所以从整体成本来看和天然气相当。由此看出,工业醇基燃料油不仅达到环保需求,还能为企业节省成本。 二.环保性能比较 因醇基燃料油主要成分为甲醇,根据化学方程式 2CH 3OH+3O 2 =2CO 2 +4H 2 O 可以看出燃烧产物只有二氧化碳和水,没有烟尘及其他残留物,减少对设备的损坏,同时从化学式可以算出燃烧一公斤醇基燃料产生约公斤二氧化碳。而燃烧1Nm3天然气产生公斤二氧化碳,燃烧一公
斤柴油产生公斤二氧化碳。按单台4吨蒸汽锅炉每天运行8小时计,具体见表2-2 表 2-2 4吨蒸汽锅炉不同燃料二氧化碳排放比较由表2-2数据可以看出,工业醇基燃料燃烧后二氧化碳排放和天然气相当,和柴油相比每年减少排放600吨,减少30%。 综合以上两方面比较可以看出,工业醇基燃料油和柴油相比,无论在经济上还是在环保性能上都具有很大的优势;而同天然气相比,在经济性上和环保性能上来看两者相当,但在安全性方面和储运以及燃料供应充足方面具有较大的优势。 工业醇基燃料油概念
制冷单位制冷量功率换算表
制冷单位制冷量换算表 第1页共4页
2008年05月28日星期三00:11 各种制冷量单位的换算关系如下: 1.1kcal/h(大卡/小时)=1.163W,1W=0.8598kcal/h; 2.1Btu/h(英热单位/小时)=0.2931W,1W= 3.412Btu/h; 3.1USRT(美国冷吨)=3.517kW,1kW=0.28434USRT; 4.1kcal/h=3.968Btu/h,1Btu/h=0.252kcal/h; 5.1USRT=3024kcal/h,10000kcal/h=3.3069USRT; 6.1匹=2.5kW(用于风冷机组),1匹=3kW(用于水冷机组) 说明: 1.“匹”用于动力单位时,用Hp(英制匹)或Ps(公制匹)表示,也称“马力”,1 Hp(英制匹)=0.7457kW,1Ps(公制匹)=0.735kW; 2.中小型空调制冷机组的制冷量常用“匹”表示,大型空调制冷机组的制冷量 常用“冷吨(美国冷吨)”表示。 冷吨通常用来标称功率较大的中央空调,一冷吨=3024大卡=3516W,计算是以大卡(KCAL/H)或瓦(W)来计算的,一般来说,1匹家用空调对应于制冷量约为2000大卡=2324W(1大卡=1Kcal/时=1.161W)。而中央空调对应的制冷系数 为4~5之间。 空调制冷量可以用匹数(PH)或冷吨(RT)来标称,匹是功率单位, 1PH=745W,如要转换成制冷量则需乘以制冷系数ε=2.8~5,冷吨(RT)是制冷量单位,冷吨通常用来标称功率较大的中央空调,一冷吨=3024大卡=3516W,计算是以大卡(KCAL/H)或瓦(W)来计算的,一般来说,1匹家用空调对应于制冷量约为2000大卡=2324W(1大卡=1Kcal/时=1.161W)。而中央空调对应的制冷系数为4~5之间 螺杆机的制冷剂充注计算(公司估算) 制冷剂(R22)Kg=(制冷量/3.516)*0.6-----0.8\\\ 空调的制冷量和功率。 常用:1050KW=300RT(冷吨)。 冷媒冲注系数:0.6-0.8。1KW=860KCal 1HP=1匹=736瓦-----745.7W=2684.52KJ 第2页共4页
电流、电压、功率的关系及公式
电流I,电压V,电阻R,功率W,频率F W=I的平方乘以R V=IR W=V的平方除以R 电流=电压/电阻 功率=电压*电流*时间 电流I,电压V,电阻R,功率W,频率F W=I的平方乘以R V=IR 电流I,电压V,电阻R,功率W,频率F W=I的平方乘以R V=IR W=V的平方除以R 电压V(伏特),电阻R(欧姆),电流强度I(安培),功率N(瓦特)之间的关系是: V=IR,N=IV =I*I*R, 或也可变形为:I=V/R,I=N/V等等.但是必须注意,以上均是在直流(更准确的说,是直流稳态)电路情况下推导出来的!其它情况不适用.如交流电路,那要对其作补充和修正求电压、电阻、电流与功率的换算关系 电流=I,电压=U,电阻=R,功率=P U=IR,I=U/R,R=U/I, P=UI,I=P/U,U=P/I
P=U2/R,R=U2/P 就记得这一些了,不知还有没有 还有P=I2R P=IU R=U/I 最好用这两个;如电动机电能转化为热能和机械能。电流 符号: I 符号名称: 安培(安) 单位: A 公式: 电流=电压/电阻I=U/R 单位换算: 1MA(兆安)=1000kA(千安)=1000000A(安) 1A(安)=1000mA(毫安)=1000000μA(微安)单相电阻类电功率的计算公式= 电压U*电流I 单相电机类电功率的计算公式= 电压U*电流I*功率因数COSΦ 三相电阻类电功率的计算公式= 1.732*线电压U*线电流I (星形接法) = 3*相电压U*相电流I(角形接法) 三相电机类电功率的计算公式= 1.732*线电压U*线电流I*功率因数COSΦ(星形电流=I,电压=U,电阻=R,功率=P U=IR,I=U/R,R=U/I, P=UI,I=P/U,U=P/I P=U2/R,R=U2/P 就记得这一些了,不知还有没有 还有P=I2R ⑴串联电路P(电功率)U(电压)I(电流)W(电功)R(电阻)T(时间)
功率、功及热量
卡路里就是热量的计算单位,1卡就是我们所吃的食物燃烧之后,使1克的水温度上升摄氏1度时的所需的热量,千卡就是使1公升的水温度上升摄氏1度所需的热量,千卡又叫做大卡。 千卡=大卡 1千卡=1000卡 1千焦=1000焦耳 1千卡/1大卡=1000卡 1千卡/1大卡=4.184千焦(kJ) 1卡=4.182焦耳 国际标准单位是千焦,而通俗我们一般讲的是大卡. 常见食品的热量表(单位大卡/克) 功的最常用单位:千克米、牛顿米、焦耳、瓦时、千瓦时、卡、千卡 1.力学:千克米、牛顿米、焦耳 1千克米=9.8牛顿米;1牛顿米=1焦耳; 2.电功:瓦时、千瓦时、度、瓦特秒 1000瓦时=千瓦时=1度;1瓦时=3600瓦特秒 3.热学:卡、千卡 1000卡=1千卡; 热功当量:1卡=4.186焦耳,1瓦秒=1焦耳 焦耳跟这FS乘积的单位有什么关系呢? 好比一个人两个名字,FS乘积的单位是牛顿米,也叫焦 瓦特是功率的单位,而度是电功的单位,它们之间不能相互转换。 如果在功率上再乘以一个时间,那么这个结果就是功。功的单位有焦耳和千瓦时,千瓦时就是平时所说的“度”,它们之间的关系如下: 1焦=1瓦·秒 1千瓦时=1千瓦·小时=1000瓦·小时=1000*3600瓦·秒=3600000焦 即: 1J=1W·s 1kWh=1kW·h=1000w·h=1000*3600w·s=3600000J 1WH=0.001度=1/1000度 ⒈电功W:电流所做的功叫电功。电流作功过程就是电能转化为其它形式的能。 公式:W=UQ W=UIt=U2t/R=I2Rt W=Pt 单位:W焦U伏特I安培t秒Q库P瓦特
单位换算(热量长度)
盛泰锅炉厂:蒸汽锅炉吨位与热水锅炉MW的关系 对于蒸汽锅炉,吨位为1t/h的蒸汽锅炉,是指每小时蒸汽的额定蒸发量为1吨,当与热水锅炉相对应的时候,一般又是指的低压锅炉且产生的是饱和蒸汽的,低压锅炉的额定工作压力一般为(表压),而锅炉的给水温度为20℃,因此对于蒸汽锅炉,当1吨的水经过吸热后,变成1吨的饱和蒸汽()时,所需吸收的热量计算方式: 饱和蒸汽()的焓值:kg (饱和蒸汽焓值查询) 20℃给水焓值约为:20×=kg 1kg水变成1kg蒸汽需要吸收的热量: 所以,1吨20℃的水变成1吨下的饱和蒸汽所需要吸收的热量:2704000kj=2704MJ=2704÷3600≈。 通常所说的,1吨蒸汽锅炉对应的热水锅炉,其实就是1吨的蒸汽锅炉与的热水锅炉,两者的传递给水(或蒸汽)的热量是相同的。 满意答案好评率:62% 1焦耳=千克·米=*10-7千瓦·小时=*10-7公制马力小时=*10-7英制马力小时=*10-4千卡=*10-4英热单位 1卡(cal)=焦耳(J) 1英热单位(Btu)=焦耳(J) 1千克力米(kgf·m)=焦耳(J) 1英尺磅力(ft·1bt)=焦耳(J) 1米制马力小时 (hp·h)=*106焦耳(J) 1英制马力小时(UKHp·h) =*106焦耳(J) 1千瓦小时(kw·h)=*106焦耳(J) 1大卡=焦耳(J) 长度 1千米(km)=英里(mile) 1米(m)=英尺(ft)=码(yd) 1厘米(cm)=英寸(in) 1英里(mile)=千米(km) 1英尺(ft)=米(m) 1英寸(in)=厘米(cm) 1海里(n mile)=千米(km) 1码(yd)=米(m) 1英尺(ft)=12英寸(in) 1码(yd)=3英尺(ft) 1英里(mile)=5280英尺(ft) 1海里(n mile)=英里(mile) 质量 1吨(t)=1000千克(kg)=2205磅(lb)= 短吨=长吨 1千克(kg)=磅(lb) 1短吨=吨(t)=2000磅(1b)