Jetcloud collision, 3D gasdynamic simulations of HH 110
华为创通 大飞机 仿真
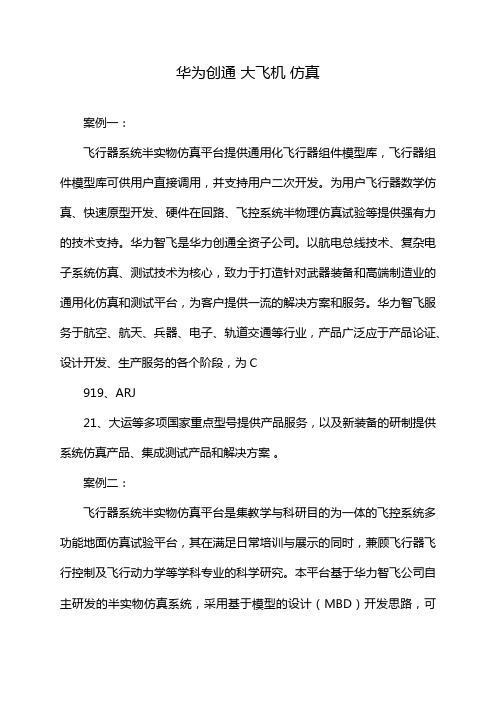
华为创通大飞机仿真案例一:飞行器系统半实物仿真平台提供通用化飞行器组件模型库,飞行器组件模型库可供用户直接调用,并支持用户二次开发。
为用户飞行器数学仿真、快速原型开发、硬件在回路、飞控系统半物理仿真试验等提供强有力的技术支持。
华力智飞是华力创通全资子公司。
以航电总线技术、复杂电子系统仿真、测试技术为核心,致力于打造针对武器装备和高端制造业的通用化仿真和测试平台,为客户提供一流的解决方案和服务。
华力智飞服务于航空、航天、兵器、电子、轨道交通等行业,产品广泛应于产品论证、设计开发、生产服务的各个阶段,为C919、ARJ21、大运等多项国家重点型号提供产品服务,以及新装备的研制提供系统仿真产品、集成测试产品和解决方案。
案例二:飞行器系统半实物仿真平台是集教学与科研目的为一体的飞控系统多功能地面仿真试验平台,其在满足日常培训与展示的同时,兼顾飞行器飞行控制及飞行动力学等学科专业的科学研究。
本平台基于华力智飞公司自主研发的半实物仿真系统,采用基于模型的设计(MBD)开发思路,可支撑飞行器数学仿真试验(MIL)、飞控算法快速控制原型开发(RCP)、飞控系统硬件在环(HIL)以及飞控系统半物理或全物理仿真试验。
平台提供飞行仿真综合控制、模型编译及下载管理、仿真数据处理等功能,为用户提供一个高易用性、高可靠性、强实时性的设计、开发、仿真、验证平台。
飞行器系统半实物仿真平台提供全机飞行器、飞控系统、惯性导航、大气机、起落架系统等仿真模型,并模拟与机载设备交联的外部环境,结合自主研发的半实物仿真平台,完成对物理效应模拟器(转台、大气物理效应仿真系统、导航模拟器等)的接联和驱动,构建完整的飞控闭环仿真系统,为机载设备的控制算法验证、设计开发和验证提供支持。
同时提供飞行仿真三维可视化视景,直观显示飞行位置、姿态及舱内环境等,并与VR技术结合实现沉浸式仿真。
深度强化学习为智能航空发动机控制赋能
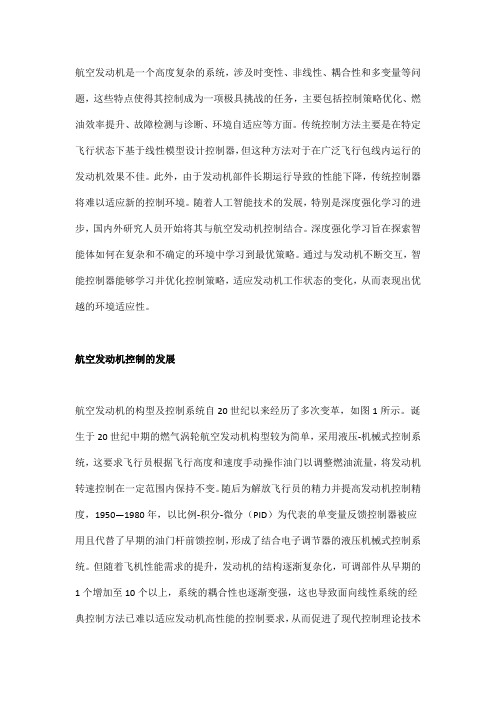
航空发动机是一个高度复杂的系统,涉及时变性、非线性、耦合性和多变量等问题,这些特点使得其控制成为一项极具挑战的任务,主要包括控制策略优化、燃油效率提升、故障检测与诊断、环境自适应等方面。
传统控制方法主要是在特定飞行状态下基于线性模型设计控制器,但这种方法对于在广泛飞行包线内运行的发动机效果不佳。
此外,由于发动机部件长期运行导致的性能下降,传统控制器将难以适应新的控制环境。
随着人工智能技术的发展,特别是深度强化学习的进步,国内外研究人员开始将其与航空发动机控制结合。
深度强化学习旨在探索智能体如何在复杂和不确定的环境中学习到最优策略。
通过与发动机不断交互,智能控制器能够学习并优化控制策略,适应发动机工作状态的变化,从而表现出优越的环境适应性。
航空发动机控制的发展航空发动机的构型及控制系统自20世纪以来经历了多次变革,如图1所示。
诞生于20世纪中期的燃气涡轮航空发动机构型较为简单,采用液压-机械式控制系统,这要求飞行员根据飞行高度和速度手动操作油门以调整燃油流量,将发动机转速控制在一定范围内保持不变。
随后为解放飞行员的精力并提高发动机控制精度,1950—1980年,以比例-积分-微分(PID)为代表的单变量反馈控制器被应用且代替了早期的油门杆前馈控制,形成了结合电子调节器的液压机械式控制系统。
但随着飞机性能需求的提升,发动机的结构逐渐复杂化,可调部件从早期的1个增加至10个以上,系统的耦合性也逐渐变强,这也导致面向线性系统的经典控制方法已难以适应发动机高性能的控制要求,从而促进了现代控制理论技术的应用,线性二次型调节器(LQR)、线性二次型高斯/回路传输恢复(LQG/LTR)及多变量鲁棒控制等的各种控制方法在1980—2010年间被广泛研究和应用。
美国针对航空发动机多变量控制开展过许多研究,其中包括利用多变量控制解决F-35战斗机短距起飞垂直降落的特殊飞行任务、为确保控制精度和发动机的最佳性能对F135发动机进行多变量控制等。
航天远景自动化建模流程

航天远景自动化建模流程English Answer:Vision: Automated Modeling for Aerospace Engineering.Introduction:The aerospace industry is experiencing a paradigm shift driven by the convergence of advanced technologies such as artificial intelligence (AI), machine learning (ML), and high-performance computing (HPC). This convergence enables the automation of complex and time-consuming engineering tasks, empowering aerospace engineers to innovate faster and more efficiently. In this context, the vision of automated modeling for aerospace engineering emerges as a critical enabler for unlocking new frontiers in aircraft design, analysis, and optimization.Challenges in Aerospace Modeling:Aerospace modeling involves a wide range of complex tasks, including:Geometry Modeling: Creating accurate and detailed representations of aircraft shapes and components.Simulation and Analysis: Conducting aerodynamic, structural, and thermal simulations to evaluate aircraft performance and safety.Optimization: Iteratively refining designs to improve efficiency, performance, and cost.Traditional approaches to these tasks are often manual, labor-intensive, and prone to errors. Automation can address these challenges by streamlining processes, reducing human intervention, and improving the accuracy and consistency of results.Benefits of Automated Modeling:Automated modeling offers numerous benefits foraerospace engineering:Increased Efficiency: Automation eliminates repetitive and time-consuming manual tasks, allowing engineers to focus on higher-value activities.Improved Accuracy: AI-powered algorithms can analyze vast amounts of data and identify subtle patterns, leading to more precise and reliable models.Reduced Costs: Automation can reduce the cost of modeling by eliminating human errors and minimizing rework.Faster Innovation: Automated modeling enables rapid design exploration and optimization, accelerating the development cycle and fostering innovation.Automation Technologies:A combination of AI, ML, and HPC technologies can be leveraged to automate aerospace modeling tasks:AI: AI algorithms can analyze data, identify patterns, and make predictions, enabling intelligent decision-making and model generation.ML: ML algorithms can learn from historical data and automatically generate models, reducing the need for manual parameter tuning.HPC: HPC platforms provide the computational power necessary to run complex simulations and optimize models efficiently.Implementation Roadmap:To realize the vision of automated modeling, a structured implementation roadmap is essential:Phase 1: Data Collection and Curation: Establish a comprehensive data repository and develop tools for data cleaning and transformation.Phase 2: Algorithm Development: Design and implementAI and ML algorithms tailored to specific aerospacemodeling tasks.Phase 3: Model Validation and Certification:Thoroughly validate and certify automated models to ensure accuracy and reliability.Phase 4: Integration and Deployment: Integrate automated modeling tools into existing engineeringworkflows and make them accessible to a wide range of users.Conclusion:Automated modeling is poised to revolutionize aerospace engineering by enabling faster, more efficient, and more accurate design and analysis. Through the strategic implementation of AI, ML, and HPC technologies, the aerospace industry can unlock new possibilities, drive innovation, and achieve unprecedented levels of performance and safety.Chinese Answer:远景,航天自动化建模流程。
航空航天工程师的航空器设计软件
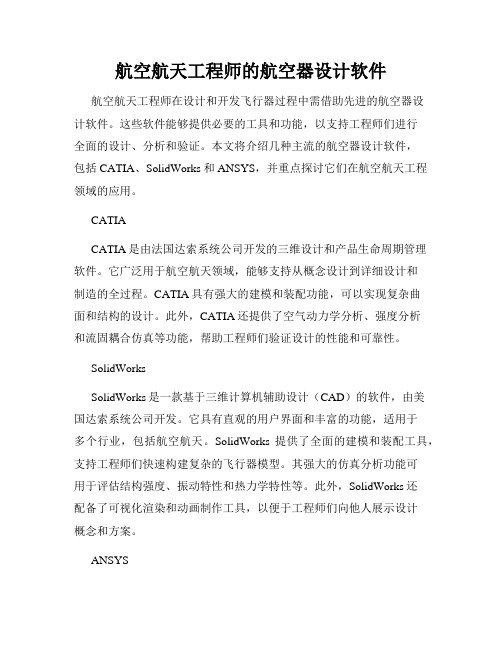
航空航天工程师的航空器设计软件航空航天工程师在设计和开发飞行器过程中需借助先进的航空器设计软件。
这些软件能够提供必要的工具和功能,以支持工程师们进行全面的设计、分析和验证。
本文将介绍几种主流的航空器设计软件,包括CATIA、SolidWorks和ANSYS,并重点探讨它们在航空航天工程领域的应用。
CATIACATIA是由法国达索系统公司开发的三维设计和产品生命周期管理软件。
它广泛用于航空航天领域,能够支持从概念设计到详细设计和制造的全过程。
CATIA具有强大的建模和装配功能,可以实现复杂曲面和结构的设计。
此外,CATIA还提供了空气动力学分析、强度分析和流固耦合仿真等功能,帮助工程师们验证设计的性能和可靠性。
SolidWorksSolidWorks是一款基于三维计算机辅助设计(CAD)的软件,由美国达索系统公司开发。
它具有直观的用户界面和丰富的功能,适用于多个行业,包括航空航天。
SolidWorks提供了全面的建模和装配工具,支持工程师们快速构建复杂的飞行器模型。
其强大的仿真分析功能可用于评估结构强度、振动特性和热力学特性等。
此外,SolidWorks还配备了可视化渲染和动画制作工具,以便于工程师们向他人展示设计概念和方案。
ANSYSANSYS是一款广泛应用于工程仿真的软件,包括结构力学、热分析、流体力学等各个领域。
在航空航天工程中,ANSYS被广泛用于飞行器的结构和气动特性分析。
它提供了高度准确的数值求解器和多种模型库,能够模拟复杂的物理现象和工程场景。
ANSYS的结构分析模块可以用于评估飞行器在各种载荷下的强度和刚度。
其气动特性分析模块则可用于评估飞行器的空气动力学性能,并优化设计以提高飞行效率和稳定性。
综合应用航空航天工程师通常会将这些航空器设计软件综合应用,以满足设计和仿真的全面需求。
首先,工程师们可以使用CATIA进行飞行器的整体设计和装配。
随后,借助SolidWorks进行细节设计和零部件的建模。
国产三维电磁仿真软件RDSim2022R1版发布

国产三维电磁仿真软件RDSim2022R1版发布RDSim2022R1 简介三维电磁仿真软件RDSim2022R1是一款由霍莱沃自主研发的全波电磁仿真平台。
本软件运用改进的矩量法、快速算法及高频算法实现高精度、高效率的电磁仿真,覆盖天线、大尺寸RCS、微波器件及天线布局等应用方向,为高频电磁问题分析提供全方位的支持。
最新版本的RDSim中开启了“云平台”线上仿真、CMA特征模分析及阵列综合优化模块,并对算法、网格剖分、材料设置等功能进行了全方位的升级。
新功能列表1. 从单机版到云平台l 用户将不再受硬件和软件条件的制约,只需接入网络,便可以通过账号登录云平台,随时随地线上仿真l 在网页中,用户可以直接进行数据分析,更好地满足数据共享与调用的需求l 云平台分为用户界面与管理界面:用户界面面向于工程师,管理界面面向于系统管理员,形成系统的分级化管理l 仿真团队可以线上建立协作工程,指派分工并共享结果数据,实现团队间高效的在线协同设计2. 高精度全波仿真算法升级l 新增体等效(VEP)积分方程求解,适用于不均匀介质、薄介质及各向异性材料l 支持分层介质结构快速求解l 提升了双稳定多层快速多极子算法收敛性,大幅度提高迭代求解效率l 快速算法可实现亿级网格求解3. 网格剖分功能升级l 新增高精度体网格剖分,适用于各类复杂体结构的精确计算l 新增网格导入与网格模型材料设置4. 阻抗边界条件设置l 用以模拟已知阻抗值的电阻性表面,高效仿真多层媒质结构l 阻抗边界支持仿真频变与非频变材料,适用于模拟多种元器件、复合电路以及复杂超材料结构5. 添加元器件功能l 元器件功能支持集成电路耦合计算,适用于仿真天线负载l 支持复合电路微波器件仿真6. 阵列综合优化l 包含多种阵列与栅格格式,一键生成自定义天线阵,大幅度节约建模时间l 专用的阵列快速仿真算法可实现大型阵列天线快速仿真需求,提高大型阵列仿真效率l 配置阵列优化与稀疏功能,以最少的单元数量和最优的天线排布达到特定阵列性能指标,全方位辅助天线阵设计与计算7. CMA特征模天线设计与优化l 具备业界领先的复杂电磁结构特征模精确高效求解技术l 可对任意结构的天线固有模式进行分析并用于天线的设计和优化l 通过分析多种天线模式得到最佳天线结构与馈电位置,实现快速天线设计l 适用于复杂环境中的天线性能分析,并在天线与环境产生耦合的情况下将天线优化至最佳工作状态。
一种基于三维烟雾的实时模拟研究
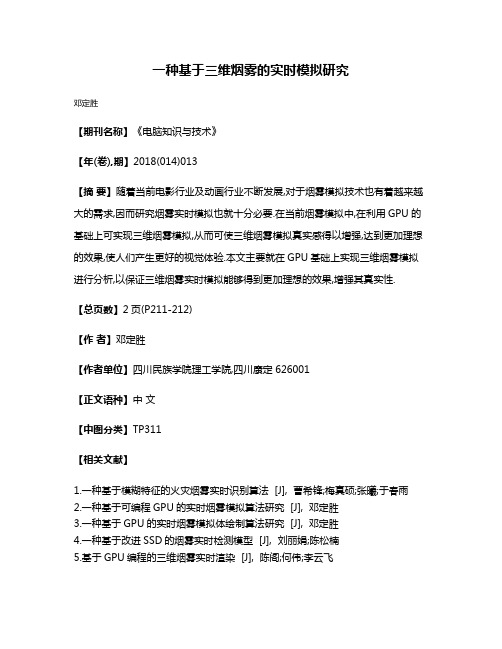
一种基于三维烟雾的实时模拟研究
邓定胜
【期刊名称】《电脑知识与技术》
【年(卷),期】2018(014)013
【摘要】随着当前电影行业及动画行业不断发展,对于烟雾模拟技术也有着越来越大的需求,因而研究烟雾实时模拟也就十分必要.在当前烟雾模拟中,在利用GPU的基础上可实现三维烟雾模拟,从而可使三维烟雾模拟真实感得以增强,达到更加理想的效果,使人们产生更好的视觉体验.本文主要就在GPU基础上实现三维烟雾模拟进行分析,以保证三维烟雾实时模拟能够得到更加理想的效果,增强其真实性.
【总页数】2页(P211-212)
【作者】邓定胜
【作者单位】四川民族学院理工学院,四川康定626001
【正文语种】中文
【中图分类】TP311
【相关文献】
1.一种基于模糊特征的火灾烟雾实时识别算法 [J], 曹希锋;梅真硕;张曦;于春雨
2.一种基于可编程GPU的实时烟雾模拟算法研究 [J], 邓定胜
3.一种基于GPU的实时烟雾模拟体绘制算法研究 [J], 邓定胜
4.一种基于改进SSD的烟雾实时检测模型 [J], 刘丽娟;陈松楠
5.基于GPU编程的三维烟雾实时渲染 [J], 陈阁;何伟;李云飞
因版权原因,仅展示原文概要,查看原文内容请购买。
国内外自动驾驶仿真软件总结
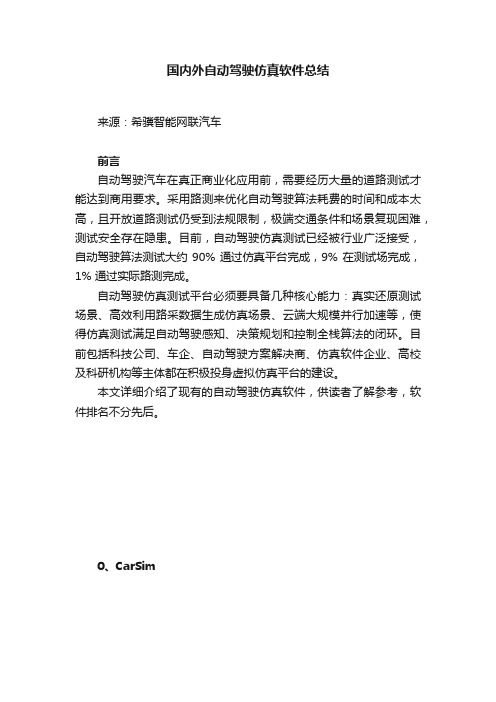
国内外自动驾驶仿真软件总结来源:希骥智能网联汽车前言自动驾驶汽车在真正商业化应用前,需要经历大量的道路测试才能达到商用要求。
采用路测来优化自动驾驶算法耗费的时间和成本太高,且开放道路测试仍受到法规限制,极端交通条件和场景复现困难,测试安全存在隐患。
目前,自动驾驶仿真测试已经被行业广泛接受,自动驾驶算法测试大约 90% 通过仿真平台完成,9% 在测试场完成,1% 通过实际路测完成。
自动驾驶仿真测试平台必须要具备几种核心能力:真实还原测试场景、高效利用路采数据生成仿真场景、云端大规模并行加速等,使得仿真测试满足自动驾驶感知、决策规划和控制全栈算法的闭环。
目前包括科技公司、车企、自动驾驶方案解决商、仿真软件企业、高校及科研机构等主体都在积极投身虚拟仿真平台的建设。
本文详细介绍了现有的自动驾驶仿真软件,供读者了解参考,软件排名不分先后。
0、CarSimCarSim,还有相关的TruckSim和BikeSim是Mechanical Simulation 公司开发的强大的动力学仿真软件,被世界各国的主机厂和供应商所广泛使用。
CarSim针对四轮汽车,轻型卡车,TruckSim 针对多轴和双轮胎的卡车,BikeSim针对两轮摩托车。
CarSim是一款整车动力学仿真软件,主要从整车角度进行仿真,它内建了相当数量的车辆数学模型,并且这些模型都有丰富的经验参数,用户可以快速使用,免去了繁杂的建模和调参的过程。
CarSim模型在计算机上运行的速度可以比实时快10倍,可以仿真车辆对驾驶员控制, 3D路面及空气动力学输入的响应,模拟结果高度逼近真实车辆,主要用来预测和仿真汽车整车的操纵稳定性、制动性、平顺性、动力性和经济性。
CarSim自带标准的Matlab/Simulink 接口,可以方便的与Matlab/Simulink进行联合仿真,用于控制算法的开发,同时在仿真时可以产生大量数据结果用于后续使用Matlab或者Excel进行分析或可视化。
基于计算流体动力学的风洞试验数据仿真研究
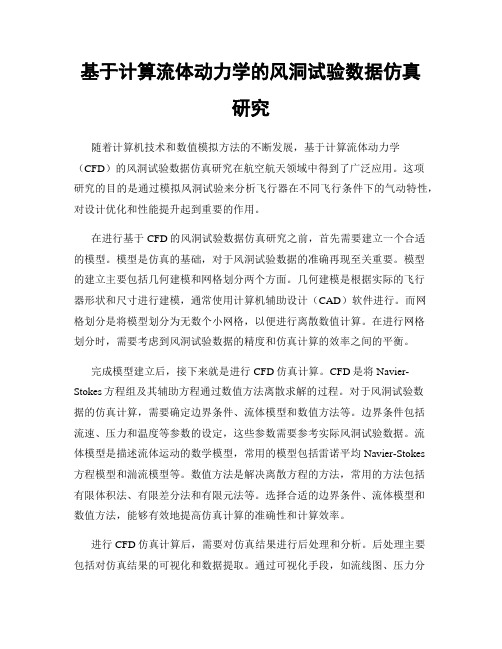
基于计算流体动力学的风洞试验数据仿真研究随着计算机技术和数值模拟方法的不断发展,基于计算流体动力学(CFD)的风洞试验数据仿真研究在航空航天领域中得到了广泛应用。
这项研究的目的是通过模拟风洞试验来分析飞行器在不同飞行条件下的气动特性,对设计优化和性能提升起到重要的作用。
在进行基于CFD的风洞试验数据仿真研究之前,首先需要建立一个合适的模型。
模型是仿真的基础,对于风洞试验数据的准确再现至关重要。
模型的建立主要包括几何建模和网格划分两个方面。
几何建模是根据实际的飞行器形状和尺寸进行建模,通常使用计算机辅助设计(CAD)软件进行。
而网格划分是将模型划分为无数个小网格,以便进行离散数值计算。
在进行网格划分时,需要考虑到风洞试验数据的精度和仿真计算的效率之间的平衡。
完成模型建立后,接下来就是进行CFD仿真计算。
CFD是将Navier-Stokes方程组及其辅助方程通过数值方法离散求解的过程。
对于风洞试验数据的仿真计算,需要确定边界条件、流体模型和数值方法等。
边界条件包括流速、压力和温度等参数的设定,这些参数需要参考实际风洞试验数据。
流体模型是描述流体运动的数学模型,常用的模型包括雷诺平均Navier-Stokes方程模型和湍流模型等。
数值方法是解决离散方程的方法,常用的方法包括有限体积法、有限差分法和有限元法等。
选择合适的边界条件、流体模型和数值方法,能够有效地提高仿真计算的准确性和计算效率。
进行CFD仿真计算后,需要对仿真结果进行后处理和分析。
后处理主要包括对仿真结果的可视化和数据提取。
通过可视化手段,如流线图、压力分布图和速度矢量图等,可以直观地观察风洞试验数据的分布情况,识别可能存在的问题。
数据提取则是将仿真结果中感兴趣的参数提取出来进行进一步的分析。
常用的数据提取手段包括平均值、谱分析和涡旋识别等。
通过后处理和分析,可以得到准确的风洞试验数据仿真结果,并对飞行器的气动特性进行全面的评估。
基于CFD的风洞试验数据仿真研究的优势主要体现在以下几个方面。
- 1、下载文档前请自行甄别文档内容的完整性,平台不提供额外的编辑、内容补充、找答案等附加服务。
- 2、"仅部分预览"的文档,不可在线预览部分如存在完整性等问题,可反馈申请退款(可完整预览的文档不适用该条件!)。
- 3、如文档侵犯您的权益,请联系客服反馈,我们会尽快为您处理(人工客服工作时间:9:00-18:30)。
a r X i v :a s t r o -p h /0206242v 1 14 J u n 2002Astronomy &Astrophysics manuscript no.MS2563February 5,2008(DOI:will be inserted by hand later)Jet/cloud collision,3D gasdynamic simulations of HH 110A.C.Raga 1,E.M.de Gouveia Dal Pino 2,A.Noriega-Crespo 3,P.D.Mininni 4,P.F.Vel´a zquez 11Instituto de Ciencias Nucleares,UNAM,Ap.70-,04510D.F.,M´e xico e-mail:raga@astroscu.unam.mx,pablo@nuclecu.unam.mx2Instituto de Astronomia,Geofisica e Ciencias Atmosfericas,Universidade de S˜a o Paulo,R.do Mat˜a o 1226,055-08-090S˜a o Paulo,SP,Brasil e-mail:dalpino@p.br3SIRTF Science Center,California Institute of Technology,IPAC 100-22,Pasadena,CA 91125,USA e-mail:alberto@ 4Departamento de F ´ısica,Facultad de Ciencias Exactas y Naturales,Universidad de Buenos Aires,Ciudad Universitaria,1428Buenos Aires,Argentina e-mail:mininni@df.uba.arAbstract.We present 3D,gasdynamic simulations of jet/cloud collisions,with the purpose of modelling the HH 270/110system.From the models,we obtain predictions of H αand H 21-0s(1)emission line maps,which qualitatively reproduce some of the main features of the corresponding observations of HH 110.We find that the model that better reproduces the observed structures corresponds to a jet that was deflected at the surface of the cloud ∼1000yr ago,but is now boring a tunnel directly into the cloud.This model removes the apparent contradiction between the jet/cloud collision model and the lack of detection of molecular emission in the crossing region of the HH 270and HH 110axes.Key words.ISM:Herbig-Haro objects —ISM:jets and outflows —ISM:kinematics and dynamics —ISM:individual (HH 110)—shock waves1.IntroductionThe Herbig-Haro (HH)jet HH 110(discovered by Reipurth &Bally 1986)is the best observed example of a possible HH jet/dense cloud collision.Reipurth et al.(1996)have interpreted the rather unique,collimated but quite chaotic structure of HH 110as the result of a de-flection of the faint HH 270jet through a collision with a dense cloud.The evidence presented by Reipurth et al.(1996)for this interpretation can be summarized as follows:–no stellar source has been detected aligned with the HH 110jet,–the HH 270jet (ejected from a detected IR and radio source,see Rodr´ıguez et al.1998)points towards the beginning of the HH 110jet,–the proper motions of HH 270and HH 110have an approximately 2to 1ratio,which is completely con-sistent with the ≈60◦deflection angle defined by the locci of the two jets (the flow approximately lying on the plane of the sky).2Raga et al.:Jet/cloud collisionsimulationsFig.1.Time sequence of the density stratifications obtained from model A.The density stratifications on the y=0 plane(which includes the outflow axis and the centre of the spherical cloud)are shown for different integration times (as indicated at the bottom left of each plot).The densities are depicted with a logarithmic greyscale,with the values given(in g cm−3)by the bar on the top left of thefigure.The x(horizontal)and z(vertical)axes are labeled in cm. very high cloud-to-jet density ratio.Raga&Cant´o(1995)suggested that this problem might be overcome if the in-cident jet did not have a completely straight jet beam,sothat the impact point would roam over the surface of thedense cloud.The regime in which the jet has punched a holethrough a cloud was described by Cant´o&Raga(1996)and Raga&Cant´o(1996).If the cloud is stratified,thepath of the jet through the cloud is curved,though thecurvature is important only if the radius of the cloud iscomparable to the jet radius.3D gasdynamic simulationsof the penetration of a jet into and through a dense,strati-fied cloud were carried out by de Gouveia Dal Pino(1999).Finally,Hurka et al.(1999)have studied the bendingof the beam of a3D MHD,non-radiative jet by a mag-neticfield with a strong gradient(as would be found atthe surface of a dense cloud).These authors show thatthis effect would help to increase the timescale over whichRaga et al.:Jet/cloud collision simulations3Fig.2.Temperature stratification (top)and adaptive grid structure (bottom)on the y =0plane obtained from model A for a t =2600yr integration time.The tempera-ture stratification is depicted with a logarithmic greyscale with the values given (in K)by the bar on the top of the figure.In the bottom plot,two thick lines separating the jet,cloud and environmental material are shown (see the text).These lines show two values of the passive scalar :ψ=0(outer contour)and ψ=1.5(inner contour).the jet/cloud surface interaction takes place,before the deflected jet is pinched off.In the present paper,we discuss 3D gasdynamic simu-lations of the interaction of a radiative jet with the surface of a dense cloud.We show the results from two simulations with different assumptions for the incident jet:Fig.3.Constant density surface (corresponding to a n =20cm −3number density of atomic nuclei)from model A for a t =2600yr integration time.The two graphs show the surface as seen from two different directions.–that the jet is ejected with a constant direction and velocity–that it is produced with a precessing outflow direction and a sinusoidally varying velocity.Through a comparison of these two simulations,we can evaluate the effect of a “roving”impact point on the pro-duction of the deflected jet.Our simulations include a treatment of the dissociation and ionization of the gas.Therefore,we can use the results to obtain predictions of atomic and molecular lines,which we directly compare with previously published images of HH 110.4Raga et al.:Jet/cloud collision simulationsIn particular,we compute predicted maps in the H2 1-0s(1)line.This is of interest because the morphology of HH110in this IR line is quite strikingly different from its morphology in atomic/ionic lines.Davis et al.(1994)and Noriega-Crespo et al.(1996)found that the H2emission is much better collimated,and lies along one of the edges of the HH110jet beam.This led Noriega-Crespo et al. (1996)to present a simple model of the molecular emission as coming from material from the dense cloud which is entrained by the jet as it brushes past the cloud surface. Our present simulations allow us to make a more definite assesment of whether or not such a mechanism actually succeeds in explaining the molecular emission of HH110.We should point out that Choi(2001)present HCO+ emission maps,in which they detect emission in the HH270/110region,but not around the“point of impact”in which the“incident”HH270jet is presumably redi-rected into the“deflected”HH110jet.This result leads them to suggest that HH110might actually not be the result of a jet/cloud collision,but that it could instead be a“straight”jet ejected from a low luminosity,undetected stellar source which is presumably more or less aligned with the direction of the HH110flow.In the conlcusions, we discuss the possible ways of reconciling the jet/cloud interaction model with the observations of Choi(2001) which are suggested by our3D gasdynamic simulations.2.The numerical simulations2.1.General featuresWe have carried out3D gasdynamic simulations of jet/cloud interactions with the yguaz´u-a adaptive grid code.This code integrates the3D(or2D)gasdynamic equations together with a set of continuity equations for atomic/ionic or chemical species.The details of the gas-dynamic and the adaptive grid algorithms have been pre-sented by Raga et al.(2000),and tests of the code are given by Sobral et al.(2000)and Raga et al.(2001).For the present simulations,we have used the follow-ing set of species:H2,H I and II,C II,III and IV,and O I,II and III(with abundances by number relative to hy-drogen:y C=6.6×10−4and y O=3.3×10−4).For the atomic/ionic reactions,we have included the collisional ionization(from Cox1970),radiative+dielectronic recom-bination(from Aldrovandi&P´e quignot1973,1976)and O/H charge exchange processes.The H2dissociation and cooling has been included in the same way as in Raga et al.(1995).The cooling associated with the atomic/ionic species has been included as described in appendix A.We have computed two jet/cloud interaction models, which share the following characteristics.In both mod-els,an initially atomic jet(except for C,which is singly ionized)of number density n j=50cm−3and temper-ature T j=1000K interacts with a spherical,homoge-neous molecular cloud(with all H in the form of H2) of number density n c=5000cm−3and T c=1K. The cloud is surrounded by a homogeneous,neutral en-vironment of density d env=10cm−3and temperature T env=1000K.Therefore,the jet to cloud(mass)density ratio isρj/ρc=1/100.In both simulations,the cloud has a r c=4×1017cm radius,and the jet has an initial,top-hat cross section of radius r j=1.5×1016cm.The jet is injected at (x,y,z)=(0,0,0)with the outflow axis in the z-direction. Free outflow conditions are applied on all of the outer boundaries of the computational domain,except for the z=0plane,on which a reflection condition is imposed outside of the injection jet cross section.The computations are carried out on a5-level,bi-nary adaptive grid(the two coarsest levels being defined over the full computational domain,see Raga et al.2000) with a maximum resolution(along the three axes)of 3.91×1015cm.The highest resolution grid is only allowed in the regions occupied either by jet or by cloud material (which are traced by advecting a passive scalar),so that the maximum resolution allowed in the environmental ma-terial is only of7.81×1015cm.We have then computed two models,one with a jet with time-independent injection conditions(model A), and one with a precessing,variable ejection velocity jet (model B).These two models are discussed in the follow-ing two subsections.2.2.Jet with time-independent injection(model A)In this simulation,the jet is injected parallel to the z-axis,with a constant v j=300km s−1injection velocity. The computational domain extends from−4.5×1017< x<0.5×1017cm,−2.5×1017<y<2.5×1017cm and 0<z<5×1017cm.The centre of the spherical cloud is placed at(x c,y c,z c)=(3.5,0,3)×1017cm,so that the jet has a glancing collision with the surface of the cloud.Figure1shows a time series(spanning an integration time of t=2400yr)of the density stratifications on the y=0plane(this plane includes the outflow axis and the centre of the dense cloud).In this time series one sees the incident jet beam(injected at the origin,and travelling along the z-axis)impinging on the surface of the dense cloud,and being deflected onto a direction towards the top left of the xz-cuts.At t=1200yr,the jet has already dug a hole into the cloud(this hole becoming deeper at later integration times),and the deflected jet beam basically becomes cut offat its base.At this time,the jet/cloud impact point lies within,rather than at the surface of the cloud.However,the material deflected by the cloud surface at earlier times continues to travel away from the cloud, leaving a complex“wake”,joining it to the point at which the incident jet impacted the cloud surface.In order to illustrate the configurations adopted by the adaptive grid,Figure2shows the temperature stratifica-tion and the adaptive grid structure on the y=0plane obtained for t=2400yr.It is clear from thisfigure that the higher resolution is not allowed on the regions occu-Raga et al.:Jet/cloud collision simulations5Fig.4.Time sequence of the density stratifications obtained from model B.The density stratifications on the y =0plane (which includes the outflow axis and the centre of the spherical cloud)are shown for different integration times (as indicated at the bottom left of each plot).The densities are depicted with a logarithmic greyscale,with the values given (in g cm −3)by the bar on the top left of the figure.The x (horizontal)and z (vertical)axes are labeled in cm.pied by environmental gas,so that the leading bow shock is only resolved at the second highest resolution level.Figure 2also shows the following.We have integrated an advection equation for a passive scalar ψ.This scalar has been given a value of ψ=1for the jet,2for the dense cloud,and −1for the surrounding environment.In the plot showing the adaptive grid,we have also drawn two contours on the stratification of the passive scalar corresponding to values ψ=0(outer contour)and ψ=1.5(inner contour).The region in between the two contourscorresponds to the jet material (which has ψ=1).From Figure 2it is then clear that the jet material occupies the injection region and the hole in the cloud,as well as a “plug”of material (at (x,y )≈(−2.5,4.5)×1017cm)with wings which extend towards the jet/cloud impact point.The region in between the wings is filled in by a tongue of cloud material which has been swept into the deflected jet flow.We find that this entrained cloud material has interesting observational properties,which are described in section 3.6Raga et al.:Jet/cloud collision simulationsThe structure of this dense tongue is more clearly seen in Figure3,which shows a constant density3D sur-face(corresponding to a n=20cm−3number density of atomic nuclei)obtained for a t=2600yr integration time.Thisfigure shows the jet penetrating into the cloud, part of the bow shock,the denser region of the deflected jet beam and the entrained molecular gas material(which forms a structure which surrounds the incident jet,and has an elongation in the direction of the deflected jet beam).2.3.Precessing,variable ejection velocity jet(model B) We have computed a second simulation,in which the ejec-tion direction precessses around the jet axis.The pre-cession cone has anα=5◦half-opening angle,and aτp=400yr precession period.Also,the jet is in-jected with a constant density(n j=50cm−3,see§2.1), but with a sinusoidally varying ejection velocity v j(t)= (300+80sin2πt/τv)km s−1,with aτv=200yr period.For this simulation,we choose a computational domain with the same extent as the one of model A along the x and y axis(but with a diferent centering:−3.5×1017< x<1.5×1017cm and−2.5×1017<y<2.5×1017cm) but with a larger,0<z<1018cm extent along the axis. The center of the spherical cloud is placed at(x c,y c,z c)= (3.5,0,6)×1017cm.We have chosen to have a larger dis-tance from the point of injection to the jet/cloud colli-sion region in order to allow the internal working surfaces of the jet(which result from the ejection velocity time-variability)to form before colliding with the dense cloud.Figure4shows a time sequence of the density strati-fications obtained on the y=0plane(which includes the precession axis and the centre of the cloud).In thisfigure, one sees the internal working surfaces whichfirst form,and then impact the surface of the dense cloud.Because of the precession in the ejection direction,the successive working surfaces impact the cloud at different points.Through a comparison with Figure1,it is clear that this effect in-creases the time that the jet takes to dig a hole into the cloud(and therefore pinching offthe deflected jet beam). In fact,for the t=3000yr time-integration shown in Figure4,the depth of the hole is still smaller than the diameter of the impinging jet(for a similar time frame obtained from model A,the depth of the hole is of ap-proximately two jet diameters,see Figure1).Similar re-sults are deduced by analyzing different y=const.cuts through the3D density stratification.Figure5shows the temperature stratification,and the grid structure on the y=0plane obtained for a t= 2500yr time-integration(equivalent results for model A are shown in Figure2).On the graph with the adaptive grid,we again show the contours that separate the jet, cloud and environmental material(for model A,see Figure 2and the discussion at the end of§2.2).It is clear that model B has a more complex structure than model A, showing a number of condensations of jet material inthe Fig.5.Temperature stratification(left)and adaptive grid structure(right)on the y=0plane obtained from model A for a t=2500yr integration time.The temperature stratification is depicted with a logarithmic greyscale with the values given(in K)by the bar on the top of thefigure. In the right hand side plot,two thick lines separating the jet,cloud and environmental material are shown(see the text).“deflectedflow”region,which correspond to the different working surfaces that have been deflected on collision with the cloud surface.Interestingly,the center of the deflected flow region isfilled with material from the molecular cloud, as is also the case for model A(see Figure2).In the following section,we present predictions of emis-sion line intensity maps carried out from the results of models A and B.These predictions can then be compared directly with the available observations of the HH270/110 system.3.Predicted intensity maps3.1.General considerationsIn order to compare the jet/cloud interaction models de-scribed in§2with the published images of the HH270/110 system,we have obtained predicted emission line maps from the models.From the computed temperature,den-sity,electron density and H ionization and molecular frac-tions,we have computed the Hαand H21-0s(1)(2.12µm) emission coefficients.For the Hαemission line coefficient,we have included the recombination cascade and the n=1→3collisional excitation(using the corresponding excitation coefficient of Giovanardi&Palla1989).The H21-0s(1)coefficientRaga et al.:Jet/cloud collision simulations7 Fig.6.Hαmaps predicted from model A,correspondingto the integration times given on the bottom left of eachframe.The maps are depicted with the greyscale givenby the bar at the top of the graph(which gives the in-tensity values in erg cm−2s−1sterad−1).The two topframes have been computed including the dust extinctionof the dense cloud(see§3.2),and the bottom frame showsa map computed without considering this extinction.Theproper motions computed from the positions of three in-tensity maxima(as measured in the t=2000and2600yrmaps)are shown in the top frame.The x(horizontal)andz(vertical)axes are labeled incm.Fig.7.Hαand H21-0s(1)intensity maps computed frommodel A for a t=2600yr integration time(see§3.2).Theleft plot shows the Hαmap in factor of2contours and theH2map in the greyscale described by the bar at the topof the plot.The right plot shows the H2map in factor of2contours and the Hαmap in gresycale(corresponding tothe bar at the top of the plot).The greyscales(given inerg cm−2s−1sterad−1by the corresponding bars)and thecontours of the intensity maps of a given line correspondto the same range of intensities.The x(horizontal)and z(vertical)axes are labeled in cm.was computed by solving the level population equationsaccording to the prescription of Draine et al.(1983,cor-rected according to Flower et al.1986).This is by nomeans the more accurate calculation of H2level popu-lations available(see,e.g.,Flower&Pineau des Forˆe ts1999),but it is appropriate given the limited accuracy ofour rather low resolution numerical simulations.We have then computed intensity maps by integratingthe emission coefficients along lines of sight.We have as-sumed that the y=0plane(which includes the axis of theincidentflow and the centre of the cloud)coincides withthe plane of the sky.This is probably a reasonable approx-imation to the orientation of the HH270/110flow,sinceit is known that both HH270and HH110approximatelylie on the plane of the sky(Reipurth et al.1986).3.2.Intensity maps predicted from model AIn Figure6,we show the Hαemission line maps predictedfrom model A(see§2.2).From the bottom plot,it is com-pletely clear that the emission is dominated by the regionin which the incident jet beam is digging into the cloud.As the optical emission maps of HH110do not show thisemitting region,we have to conclude that it has to beabsorbed by the dust in the dense cloud(at least,if webelieve in the jet/cloud interaction model for this object).In order to illustrate the effect on the Hαmaps of suchan extinction,we have computed emission maps includingthis effect(top two maps offigure6).We have computed8Raga et al.:Jet/cloud collision simulationsthe maps assuming that the dust extinction coefficient is κd=10−20(n H/cm−3)cm−1,giving aτd=40optical depth through the radius of the cloud.We should note that if we use the standard,κd= 10−21(n H/cm−3)cm−1dust absorption coefficient,our cloud would have aτd=4central optical depth.The region on the edge of the cloud into which the jet is pen-etrating would then have a low optical depth,producing little extinction of the emission from the impact region. This,however,is not a major problem given the fact that the dense cloud present in the HH270/110region is by no means either spherical or homogeneous,and could easily produce a large extinction towards the current jet/cloud impact region(which would lie within the cloud).The Hαmaps obtained from model A(for integra-tion times t=2000and2600yr)computed with the dust extinction as described above are shown in the two top frames of Figure6.It is clear that these maps do present a qualitative similarity to HH110(see,e.g.,Reipurth et al.1996).In agreement with the observations,the emitting re-gion starts with a bright rim(in contact with the sur-face of the cloud,seefigure6)which points to a broader structure(with a complex structure of curved ridges)at larger distances from the impact region.Also,there is a faint emission halo extending parallel to the main emis-sion structure on the side directed away from the dense cloud.This is also in qualitative agreement with the Hαmaps of HH110(Reipurth et al.1996).From the two time frames shown in Figure6,we have computed proper motions for the three main intensity maxima seen in the maps.We have also computed proper motions for some of the local maxima in the region in which the emission is in contact with the cloud surface, but the resulting velocities lie between3and8km s−1, and have not been plotted in Figure6.The proper motions of the knots farther away from the cloud(shown infigure6)have values of15to45km s−1. These proper motions are substantially lower than the ones measured for the HH110knots,as Reipurth et al. (1996)have found values ranging from35to150km s−1.In Figure7,we show a comparison between the Hαand the H21-0s(1)intensity maps obtained for t=2600yr. For computing the H2map,we have considered an extinc-tion equal to1/10of the visual extinction.This results in only small optical depths towards the current jet/cloud impact region,so that the emission from this region is clearly visible.The H2emission in the base of the deflected jet re-gion is much more concentrated towards the surface of the cloud than the Hαemission.This result is in clear qualitative agreement with the morphology observed in HH110(Davis et al.1994;Noriega-Crespo et al.1996). At larger distances along the deflected jetflow,the H2 and Hαemission show spatially coincident condensations, again in agreement with the observations of HH110.3.3.Intensity maps predicted from model BIn Figure8,we show the Hαintensity maps computed from model B for t=2200and2400yr integration times, and the H21-0s(1)map obtained for t=2400yr.Because of the fact that the jet/cloud impact region still lies on the surface of the cloud,the effect of the extinction due to the dust present in the cloud is not important,and has not been included.However,the extinction would be important for maps computed for different orientations of the jet/cloud structure with respect to the plane of the sky.As a result of the precession and ejection velocity time variability of the incident jet(see§2.3),the emission maps show more complex structures than the ones obtained from model A.In particular,one can clearly see the emis-sion from bow shocks around dense“bullets”,which re-sult from the successive internal working surfaces present in the incident jet.Even though the morphology observed in the emission line maps of HH110is very complex(see, e.g.,Reipurth et al.1996),it does not appear to have such bow shock structures.Actually,the intensity maps pre-dicted from model A do resemble the structure of HH110 in a more convincing way.An interesting feature of model B is that the proper motions of the different intensity maxima(obtained by comparing the t=2200and2400yr Hαintensity maps, seefigure8)have values of close to100km s−1.These velocities are in better agreement with the ones measured for HH110than the ones obtained from model A(see§3.2 and Reipurth et al.1996).4.ConclusionsWe have presented two jet/cloud collision3D gasdy-namic simulations:one with an incident jet with time-independent injection conditions(model A),and a second one with a variable velocity,precessing incident jet(model B).Aρc/ρj=100cloud to initial jet density ratio has been chosen for both models.Model A produces a deflection of the jet beam only for a∼500-1000yr timescale,after which the incident jet starts digging a straight tunnel through the dense cloud.At later times,the deflected jet material contin-ues to travel away from the impact region,leaving behind a complex“wake”.Model B produces a broader jet/cloud impact region as a result of the jet precession.This effect results in a longer timescale for the duration of the jet deflection on the cloud surface(in fact,the jet deflection is still occuring at the end of our t=3000yr numerical simulation).Model B is more successful at reproducing the proper motions of HH110,giving∼100km s−1velocities for the Hαintensity maxima along the deflected jet beam. Model A gives velocities of∼15-45km s−1,which are substantially lower than the proper motion velocities of HH110(see Reipurth et al.1996).Raga et al.:Jet/cloud collision simulations9Fig.8.Intensity maps obtained from model B (see §3.3).The left and central frames show the H αmaps obtained for two integration times (given on the bottom left of each frame).The right hand side frame shows the H 21-0s(1)map obtained for t =2400yr.The maps are depicted with the greyscales given (in erg cm −2s −1sterad −1)by the bars on the top of each frame.The left plot shows the proper motions of several intensity maxima,computed from the t =2200and 2400yr H αintensity maps.The x (horizontal)and z (vertical)axes are labeled in cm.This difference between model A and model B is due to two effects.The first effect is that in model A,the jet is deflected only for a ∼500-1000yr timescale,and that this deflected jet material then slows down as it interacts with surrounding,environmental gas.In model B,succes-sive deflected “bullets”(i.e.,internal working surfaces)travel into the low density region left behind by the pas-sage of the head of the deflected jet,and do not interact directly with the higher density environment.The second effect is that because of the precession of model B,some of the bullets have trajectories which are more tangential to the surface of the molecular cloud.These more tangential bullets are less deflected,and therefore preserve larger ve-locities than the ones that have a more normal incidence on the cloud surface (or than the deflected jet of model A).However,in most other counts,model A is more suc-cessful than model B at reproducing the observations of HH 110:–the general qualitative appearance of the H αmaps is in better agreement with the HH 110H αimages,–the features of the H 21-0s(1)emission and their spa-tial relation to the H αemission also are in good qual-itative agreement with HH 110,–the maps that better resemble HH 110correspond to times (e.g.,the t =2400yr frame of figures 1and 6)in which the impact region is already immersed within the cloud.This last feature offers an interesting way of reconciling the jet/cloud collision model with the HCO +observations of Choi (2001).In these observations,no HCO +emission was detected in the region in which the axis of the “incident”HH 270jet crosses the axis of the “deflected”HH 110.Choi (2001)noted that this appeared to be in disagreement with a jet/cloud collision model for this system,as the cloud shock produced in the impact region should indeed pro-duce HCO +emission.The situation found in model A,however,could indeed be in agreement with the observations of Choi (2001).In this model,the impact region does not lie in the point in which the incident and deflected jet axes cross,but is instead located further along the axis of the incident jet.Interestingly,Choi (2001)does find substantial HCO +emission West of HH 110,approximately aligned with HH 270.As we have discussed in §3.2,the fact that the impact region is not observed optically can in principle be a result of the dust extinction in the dense cloud.Interestingly,some H 21-0s(1)emission is apparently detected to the West of HH 110(more or less aligned with HH 270,see Noriega-Crespo et al.1996),which in principle might be associated with the impact region.To conclude,we note the two main features of our re-sults :。