二氧化钛的紫外拉曼光谱研究外文翻译
二氧化钛纳米晶薄膜的吸收光谱和激发发射光谱(英文)
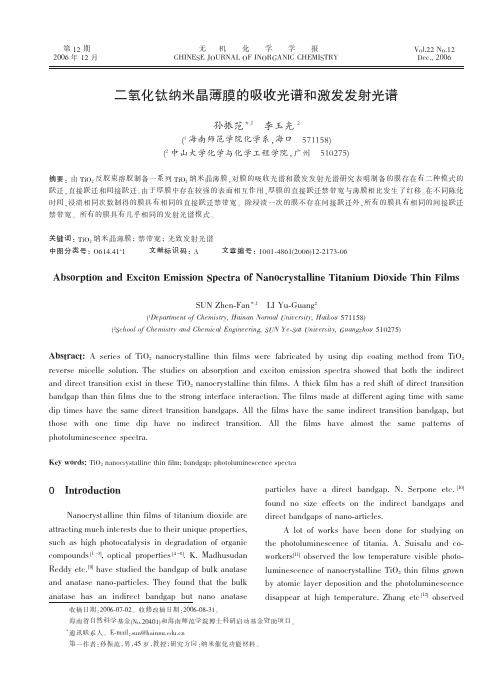
T5LO69P70 45U7 5 86L79O S528K5T, *, B7LT+27 7O9, Z(-\ M+128 2+ 06a7 7MM79O0 +2 O47 6286L79O S528K5T0 528 86L79O S528K5T0 +M 252+#5LO69P70, ? P+O +M R+LW0 45U7 S772 8+27 M+L 0O18N62K +2 O47 T4+O+P1Q627097297 +M O6O5265, ?, B1605P1 528 9+# R+LW7L0 Z((\ +S07LU78 O47 P+R O7QT7L5O1L7 U606SP7 T4+O+# P1Q627097297 +M 252+9LN0O5PP627 ;6<A O462 M6PQ0 KL+R2 SN 5O+Q69 P5N7L 87T+06O6+2 528 O47 T4+O+P1Q627097297 8605TT75L 5O 46K4 O7QT7L5O1L7, D452K 7O9 Z(A\ +S07LU78
Y*=D;
_‘a */<=." 0B #4. #*#<&*< B*,/"
"
/$,0+), *(1 1&,-0,,&.(
三种不同银纳米粒子SERS基底比较研究
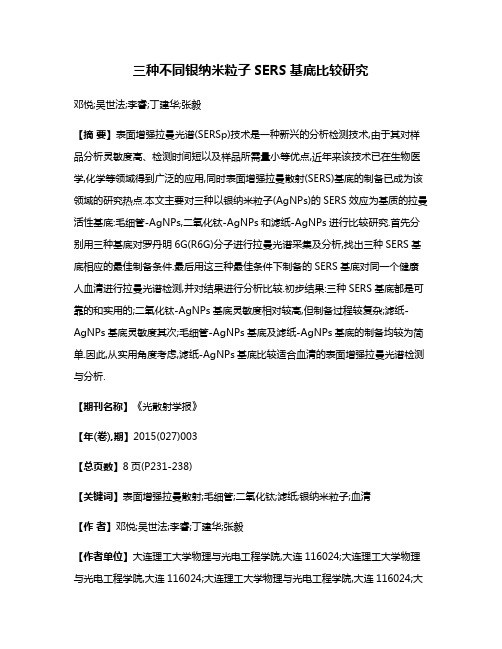
三种不同银纳米粒子SERS基底比较研究邓悦;吴世法;李睿;丁建华;张毅【摘要】表面增强拉曼光谱(SERSp)技术是一种新兴的分析检测技术,由于其对样品分析灵敏度高、检测时间短以及样品所需量小等优点,近年来该技术已在生物医学,化学等领域得到广泛的应用,同时表面增强拉曼散射(SERS)基底的制备已成为该领域的研究热点.本文主要对三种以银纳米粒子(AgNPs)的SERS效应为基质的拉曼活性基底:毛细管-AgNPs,二氧化钛-AgNPs和滤纸-AgNPs进行比较研究.首先分别用三种基底对罗丹明6G(R6G)分子进行拉曼光谱采集及分析,找出三种SERS基底相应的最佳制备条件.最后用这三种最佳条件下制备的SERS基底对同一个健康人血清进行拉曼光谱检测,并对结果进行分析比较.初步结果:三种SERS基底都是可靠的和实用的;二氧化钛-AgNPs基底灵敏度相对较高,但制备过程较复杂;滤纸-AgNPs基底灵敏度其次;毛细管-AgNPs基底及滤纸-AgNPs基底的制备均较为简单.因此,从实用角度考虑,滤纸-AgNPs基底比较适合血清的表面增强拉曼光谱检测与分析.【期刊名称】《光散射学报》【年(卷),期】2015(027)003【总页数】8页(P231-238)【关键词】表面增强拉曼散射;毛细管;二氧化钛;滤纸;银纳米粒子;血清【作者】邓悦;吴世法;李睿;丁建华;张毅【作者单位】大连理工大学物理与光电工程学院,大连116024;大连理工大学物理与光电工程学院,大连116024;大连理工大学物理与光电工程学院,大连116024;大连理工大学物理与光电工程学院,大连116024;大连理工大学物理与光电工程学院,大连116024【正文语种】中文【中图分类】O433.4拉曼光谱技术是以拉曼效应为基础建立起来的以光子作探针、具有实时检测特点的分子结构表征技术,而表面增强拉曼散射是由于金属表面局域等离子体激元共振引起的电磁增强,以及金属纳米结构和吸附分子之间相互作用引起的化学增强共同作用导致的散射增强现象,是一种非常有效的检测拉曼信号的技术,在光谱分析、生物医学等领域有着广泛的应用。
N掺杂文献翻译
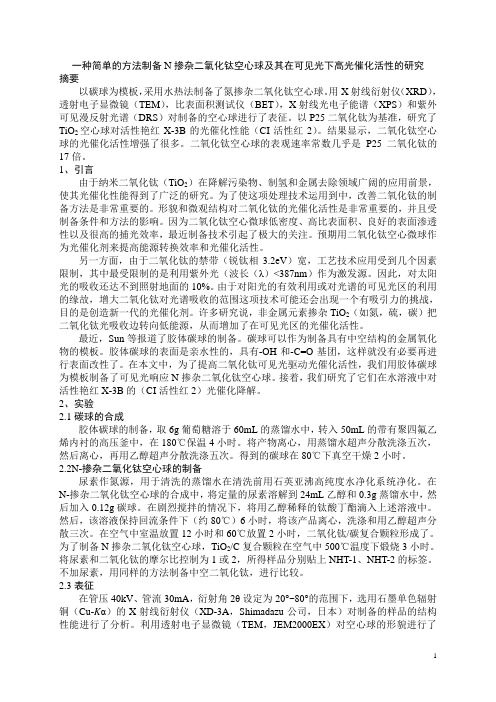
一种简单的方法制备N掺杂二氧化钛空心球及其在可见光下高光催化活性的研究摘要以碳球为模板,采用水热法制备了氮掺杂二氧化钛空心球。
用X射线衍射仪(XRD),透射电子显微镜(TEM),比表面积测试仪(BET),X射线光电子能谱(XPS)和紫外可见漫反射光谱(DRS)对制备的空心球进行了表征。
以P25二氧化钛为基准,研究了TiO2空心球对活性艳红X-3B的光催化性能(CI活性红2)。
结果显示,二氧化钛空心球的光催化活性增强了很多。
二氧化钛空心球的表观速率常数几乎是P25二氧化钛的17倍。
1、引言由于纳米二氧化钛(TiO2)在降解污染物、制氢和金属去除领域广阔的应用前景,使其光催化性能得到了广泛的研究。
为了使这项处理技术运用到中,改善二氧化钛的制备方法是非常重要的。
形貌和微观结构对二氧化钛的光催化活性是非常重要的,并且受制备条件和方法的影响。
因为二氧化钛空心微球低密度、高比表面积、良好的表面渗透性以及很高的捕光效率,最近制备技术引起了极大的关注。
预期用二氧化钛空心微球作为光催化剂来提高能源转换效率和光催化活性。
另一方面,由于二氧化钛的禁带(锐钛相3.2eV)宽,工艺技术应用受到几个因素限制,其中最受限制的是利用紫外光(波长(λ)<387nm)作为激发源。
因此,对太阳光的吸收还达不到照射地面的10%。
由于对阳光的有效利用或对光谱的可见光区的利用的缘故,增大二氧化钛对光谱吸收的范围这项技术可能还会出现一个有吸引力的挑战,目的是创造新一代的光催化剂。
许多研究说,非金属元素掺杂TiO2(如氮,硫,碳)把二氧化钛光吸收边转向低能源,从而增加了在可见光区的光催化活性。
最近,Sun等报道了胶体碳球的制备。
碳球可以作为制备具有中空结构的金属氧化物的模板。
胶体碳球的表面是亲水性的,具有-OH和-C=O基团,这样就没有必要再进行表面改性了。
在本文中,为了提高二氧化钛可见光驱动光催化活性,我们用胶体碳球为模板制备了可见光响应N掺杂二氧化钛空心球。
纳米tio2做紫外谱的步骤
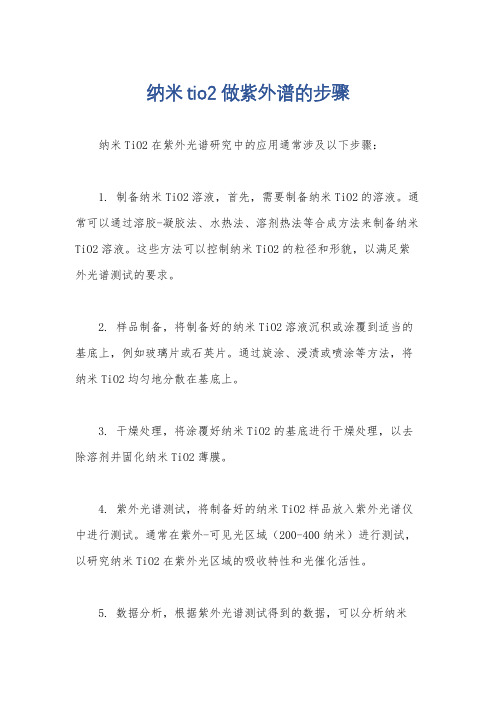
纳米tio2做紫外谱的步骤
纳米TiO2在紫外光谱研究中的应用通常涉及以下步骤:
1. 制备纳米TiO2溶液,首先,需要制备纳米TiO2的溶液。
通常可以通过溶胶-凝胶法、水热法、溶剂热法等合成方法来制备纳米TiO2溶液。
这些方法可以控制纳米TiO2的粒径和形貌,以满足紫外光谱测试的要求。
2. 样品制备,将制备好的纳米TiO2溶液沉积或涂覆到适当的基底上,例如玻璃片或石英片。
通过旋涂、浸渍或喷涂等方法,将纳米TiO2均匀地分散在基底上。
3. 干燥处理,将涂覆好纳米TiO2的基底进行干燥处理,以去除溶剂并固化纳米TiO2薄膜。
4. 紫外光谱测试,将制备好的纳米TiO2样品放入紫外光谱仪中进行测试。
通常在紫外-可见光区域(200-400纳米)进行测试,以研究纳米TiO2在紫外光区域的吸收特性和光催化活性。
5. 数据分析,根据紫外光谱测试得到的数据,可以分析纳米
TiO2在紫外光区域的吸收峰位、吸光度等参数,从而评估纳米TiO2在紫外光谱方面的性能和应用潜力。
需要注意的是,在进行纳米TiO2的紫外光谱研究时,还需要考虑样品制备的均匀性、基底对测试结果的影响等因素,以确保测试结果的准确性和可靠性。
同时,实验过程中应严格遵守相关安全操作规程,确保实验操作的安全性。
Al2O3或SiO2负载钒氧化物催化剂的紫外-拉曼光谱比较研究

3 6 工 业 催 化 2 0 0 8年第 1 0期
3 - 5 ] 液一步浸 渍 于 载 体 上 [ , 制备系列 V A l O m/ 2 3和
特征峰相对强度缓慢降低, 与V —O —V的 δ振动和 V —O —V桥键相关联分别位于 3 2 3c m-1和 4 7 4c m-1 的两个特征峰也呈现大致相同的变化趋势。与紫外 拉曼信号强度最大时的负载量区域相对应, 此时表 面负载钒 物 种 接 近 于 铺 满 一 个 单 层, 聚合态 V — O —V伸缩 振 动 特 征 峰 最 强。随 着 负 载 量 继 续 增 加, 表面单层之上形成了体相的 V O 一方面 2 5 晶体, 掩盖了下 层 的 负 载 钒 物 种, 另一方面这种体相的 V O 导致拉曼信号强 2 5 对拉曼信号存在一定的吸收, 度的降低。随着聚合态 V —O —V伸缩振动的特征 峰从8 3 4c m-1逐渐向高波数方向移动, V A l O 2 0/ 2 3催 5 2c m-1。这是由于随着钒负载量的 化剂达到了 9 增加, 单层聚合的 V O 表面负载的 x y 物种逐渐减少, 单聚钒物种逐渐向多聚物种转变。在高钒负载量时 9 9 5c m-1处 V 说明催化 O 伸缩振动峰开始出现, 剂中已经有晶相的 V O 2 5 形成。 S i O 可用于与负载氧化物 2 是一类特殊的载体, 物种成键的表面羟基密度很低, 因此, 在S i O 2 载体 和负载物种之间的相互作用非常弱, 而且 S i O 2 载体 本身的物理性能使得大多数金属氧化物在 S i O 2表 面上无法形成一种“ 浸润” 状态, 轻微的热处理足以 使这些 S i O 甚 2 表面物种迁移到其他的氧化物表面, 至在自身体相氧化物表面发生聚集。所以, 在低表 面活性的 S i O 2 载体表面很难生成完整的表面负载 单层氧化物物种, 即使借助一些特殊的手段可以得 到一些高覆盖度的 S i O 但是覆盖度较 2 负载物种, 低。在氧化铝等一般的氧化物载体之上, 氧化钒物 种在低负载量时以孤立的四配位 V O 在 4 物种为主, 高负载量( 单层以下) 时以六配位 V O 然 4 物种为主, 而在 S i O O 2 载体上仅以孤立的 V 4 物种存在。 图 2为不同负载量的 V S i O m/ 2 催化剂的紫外 - 拉曼光谱。
专业英语+二氧化钛+英文文献+翻译

二氧化钛纳米颗粒对废水脱氮除磷的效果以及对活性污泥中细菌群落改变的长期影响熊正1陈银光* 吴瑞2同济大学环境科学与工程学院污染控制与资源再生国家重点实验室,中国上海市四平路1239号,200092摘要:二氧化钛纳米颗粒〔TiO2NPs〕在很多领域的广泛应用引起了人们对其在环境中的潜在影响问题的关注。
然而,人们在TiO2NPs对生物脱氮除磷以及活性污泥中细菌群落影响的领域的调查研究确是很少的。
本实验是针对TiO2NPs在厌氧序批式反应器处于低溶解氧〔0.15—0.50mg/L〕环境下对于生物营养物质去除率的影响的评价。
研究发现,1—50mg/L浓度的TiO2NPs在与废水经过短期接触〔1天〕的情况下,对水体脱氮除磷不具有明显的效果。
然而,研究观察显示,50mg/L浓度〔高于其在环境中的相关浓度〕的TiO2NPs却使水体总氮的去除率出现明显的下降,在与废水经过长期接触反应〔70天〕后,总氮的去除率从80.3%降到24.4%,反之,生物除磷效率却没有受到影响。
变性梯度凝胶电泳图谱显示50mg/L 浓度的TiO2NPs明显减少了活性污泥中的微生物种群多样性。
荧光原位杂交分析结果说明,大量的硝化细菌,尤其是氨氧化菌在TiO2NPs与废水充分接触反应后出现急剧死亡,这也就是氨氧化菌急剧退化死亡的主要原因。
进一步的研究显示,50mg/L浓度的TiO2NPs在与废水长期充分接触之后抑制了氨单氧酶和亚硝酸盐氧化复原酶的活性,但是却对外切聚磷酸酶和多聚磷酸盐激酶没有明显的影响。
同时,TiO2NPs对于细菌细胞中的聚羟基脂肪酸酯和糖原的转变的影响也与实验所观察到的生物脱氮除磷的影响规律保持一致。
引言纳米材料因其特殊的物理和化学性质而被广泛的应用在大量的工业生产和消费产品中。
[1]特别是TiO2NPs被广泛的应用在了催化剂、遮光剂和水处理工艺中。
[2]这些TiO2NPs的广泛应用无疑会在环境中产生残留。
最近,人们在土壤、地表水、排污废水以及城市污泥中均发现有TiO2NPs的存在。
催化材料的紫外拉曼光谱研究

第30卷 第8期 催 化 学 报 2009年8月V ol. 30 No. 8Chinese Journal ofCatalysi s August 2009收稿日期: 2009-06-04. 第一作者: 范峰滔, 男, 1981年生, 博士研究生. 共同第一作者: 徐 倩, 女, 1982年生, 博士研究生. 联系人: 李 灿. Tel: (411)84379070; Fax: (411)84694447; E-mail: canli@基金来源: 国家自然科学基金 (20773118, 20673115); 国家重点基础研究发展计划 (973 计划, 2003CB615806, 2004CB720607, 2005CB221407).Edited by Foxit ReaderCopyright(C) by Foxit Software Company,2005-2008For Evaluation Only.718 催化学报第30卷surface phase. For example, UV Raman spectroscopic study clearly demonstrates that the generation of surface-phase junction on TiO2 cata-lyst can significantly enhance the photocatalytic activity for hydrogen production.Key words: UV Raman; in situ Raman; microporous and mesoporous material; transition metal-containing microporous and mesoporous material; synthesis mechanism; phase transformation催化科学与技术的发展与催化研究方法的发展是密不可分的.特别是在催化新材料和新反应的不断探索过程中,新表征技术起着很重要的作用.在过去几十年中,特别是自20世纪60年代以来,催化研究领域发展了一系列新的表征方法,几乎利用了现代科学发展所派生出来的所有新技术,包括基于光、声、电、磁、电子、原子、离子和热的原理的现代物理技术.拉曼光谱的发现距今已有80余年,激光技术的兴起使拉曼光谱成为激光分析中最活跃的研究领域之一.激光拉曼和红外光谱相辅相成,成为进行分子振动和分子结构鉴定的有力工具.近年来,随着材料科学、激光和同步加速器技术以及纳米技术的重大进展,拉曼光谱在催化研究等领域应用的机会和可能性越来越多.尤其在催化领域的研究中,拉曼光谱在担载型金属氧化物、分子筛、原位反应和吸附等研究中取得了丰硕的成果.拉曼光谱之所以在催化研究的应用中发展迅速,主要有如下几个方面的原因:①拉曼光谱能够提供催化剂本身以及表面上物种的结构信息,这是认识催化剂和催化反应最为重要的信息;②拉曼光谱较容易实现原位条件(高温、高压、复杂体系)下的催化研究;③拉曼光谱可用于催化剂制备的研究,特别是可以对催化剂制备过程进行从水相到固相的实时研究,这是许多其它光谱技术难以做到的;④近年来随着探测器灵敏度的大幅度提高和光谱仪的改进,拉曼光谱仪的信噪比大大提高.但拉曼光谱在应用中也存在着一些困难,其中荧光干扰和灵敏度较低是阻碍其广泛应用的最主要的问题.近年来发展起来的紫外拉曼光谱技术有效地解决了催化研究中所遇到的上述问题,大大扩展了拉曼光谱的应用范围.本文着重介绍了我们研究组在利用紫外拉曼以及共振拉曼技术表征含过渡金属的微孔和介孔材料中活性位结构方面取得的进展,以及最近发展起来的一种适用于原位紫外拉曼光谱研究水热反应的装置及其在研究分子筛合成机理中的应用.此外,对于氧化锆、氧化钛以及钼酸铁等在紫外区有强吸收的材料的表面相变以及表面相组成的研究,紫外拉曼光谱也显示了其独特的优势,特别是对半导体材料的光催化性能研究具有很大的优势.1含过渡金属物种的微孔-介孔分子筛材料的紫外拉曼光谱表征分子筛是一类广泛应用于工业的催化剂和催化材料[1].其中微孔分子筛由于其规则的三维孔道结构常被用于裂解、异构化、烷基化、聚合、脱氢、羰基化和芳构化等重要的工业催化过程.介孔材料由于其高的比表面积、规则的孔道结构和较大的可调节孔径(2~50nm),且传质性能优于微孔分子筛,因而成为固载催化剂的理想载体[2].将微孔分子筛和介孔分子筛材料中的硅元素和铝元素用其它元素(特别是过渡金属元素)同晶取代,可以有效地改变分子筛材料的物理化学性质[3].过渡金属取代的微孔和介孔材料,例如Fe-ZSM-5,TS-1和Ti-MCM-41,在选择性催化反应中已显示出独特的性能[1,4~8].过渡金属取代的微孔和介孔分子筛材料的性质往往取决于过渡金属的组分及结构.所以,研究分子筛材料中含过渡金属活性位的结构以及分子筛合成机理将有助于发展新型的更高活性和选择性的分子筛基催化剂[9].许多表征手段曾被用来研究过渡金属杂原子分子筛中过渡金属物种的结构和价态等信息,例如穆斯堡尔谱、X射线光电子能谱(XPS)、扩展X射线吸收精细结构(EXAFS)和紫外-可见吸收光谱等[10~16].然而,由于分子筛体系中能够引入的杂原子的含量一般很低,因此很难得到杂原子的相关信息.只有对杂原子分子筛进行大量的表征之后,通过综合分析才能得到初步的结果[3].拉曼光谱技术能够提供物质的结构信息,因此是一种潜在的、强有力的表征微孔和介孔材料的手段.但对于传统拉曼光谱技术,激发光的光源通常位于可见区,而大多数物质的荧光也处于可见区,所以荧光干扰是谱图采集过程中一个很难避免的问题.特别是微孔分子筛和介孔材料,它们往往含有有机模板剂、杂质以及表面缺陷等物种,这些物种经过激第8期 范峰滔 等: 催化材料的紫外拉曼光谱研究 719光光源照射后会发出非常强的荧光, 严重干扰拉曼光谱的收集, 从而使常规拉曼光谱很难用于分子筛材料的表征.如果能将激发光从可见区移到紫外区, 就有可能成功避开荧光的干扰 (见图 1(a)). 这是因为荧光通常出现在 300~700 nm 区域或者更长波长区域, 而在紫外区的某一波长以下荧光极少出现. 因此, 对于许多在可见拉曼光谱中存在强荧光干扰的物质, 例如氧化物和积碳等, 通过紫外拉曼光谱技术就可以成功地避开荧光干扰, 从而得到信噪比较高的拉曼光谱. 图 1(b) 给出了 AlPO-5 在 244, 325 和 532 nm 激发下的拉曼光谱图. 由图可以看出, 当激发线位于 532 nm 时, 拉曼光谱被强荧光所覆盖. 将激发线蓝移至 325 nm 时, 荧光信号大大减弱, 但此时仍然存在一定的荧光背景. 而当激发线继续蓝移至 244 nm 时, 荧光已经完全消失, 得到了信噪比非常高的 AlPO-5 的拉曼光谱. 从这个例子可以看出, 紫外共振拉曼光谱技术由于能避开荧光, 可以成功用于微孔和介孔分子筛材料的表征. 此外, 不同的分子筛有着其特征的拉曼谱峰, 通过紫外拉曼光谱可以准确地分析、鉴别不同类型的分子筛[17].当激发光波长更短时, 拉曼散射界面更大, 因此紫外拉曼光谱的灵敏度远远高于可见或近红外拉曼光谱.紫外拉曼光谱技术的另一个突出特点是, 当将紫外拉曼用于表征分子筛体系时, 由于一些组分在紫外区有明显的吸收, 紫外光可以选择性地激发这些组分相应的信息, 从而使与这些组分相关的拉曼信号大大增强, 得到共振拉曼光谱. 如图 2(b) 所示,位于 250 和 400 nm 的吸收带分别对应于两种不同组分 X 和 Y. 250 和 450 nm 激发光分别位于这两个吸收带的中心附近 (图 2(c)). 根据共振拉曼原理, 当选择 250 nm 作为拉曼光谱激发光时, X 组分相关的拉曼谱峰的强度会显著增强, 而当选择 450 nm 激发光时, Y 组分相关的拉曼谱峰的强度会显著增强. 相对于普通拉曼 (非共振拉曼), 共振拉曼光谱的强度可以增大几个数量级. 这对于研究杂原子分子筛而言非常重要. 由于分子筛体系中位于骨架或半骨架位的过渡金属杂原子物种相应的骨架氧到过渡金属之间的电荷跃迁一般位于紫外区, 例如 Fe-ZSM-5 位于 250 nm, TS-1 位于 220 nm, V-MCM-41 位于 280 nm, 因此基于共振拉曼光谱原理, 选择合适的紫外激发光共振激发这些过渡金属物种, 就可以得到其特征拉曼光谱信息. 利用紫外共振拉曼光谱的这些优点可以鉴定分子筛材料中高度隔离的骨架或半骨架过渡金属物种. 所以, 紫外共振拉曼光谱技术是一种非常重要的可用来表征分子筛材料中过渡金属物种的表征手段.1.1 TS-1 和 Ti-MCM-41 中骨架 Ti的鉴定 含 Ti 分子筛材料, 如微孔 TS-1 和 TS-2 以及介孔 Ti-MCM-41, Ti-HMS 和 Ti-MCM-48, 具有很好的选择氧化的特性[1,5~8],因此无论在工业上还是在学术上都引起了人们极大的研究热情. 在这些含 Ti 分子筛材料中, TS-1 分子筛利用过氧化氢为氧化剂在温和条件下可以将多种有机物选择氧化, 并且表现出很高的活性和选择性, 例如苯酚羟基化反应和仲醇氧化为酮的反应. 一般来讲, TS-1 分子筛中骨架 TiWavelength (nm)200300400500600700800Raman shift (cm -1)5001000图 1 荧光对常规拉曼光谱的干扰 (a) 及 244, 325 和 532 nm 激发下 AlPO-5 的拉曼光谱 (b)Fig. 1. Raman signal usually obscured by the strong fluorescence interference (a) and Raman spectra of AlPO 4-5 excited at 244, 325, and 532 nm (b).720 催 化 学 报 第30卷被认为是选择氧化的活性物种[18], 因此在选择氧化过程中, Ti–O–Si 扮演着至关重要的角色. 然而, 尽管多种表征手段被用来表征 TS-1 中的骨架 Ti, 骨架 Ti 物种的准确鉴定仍然是一个极具挑战性的课题[19~22].图 3(a)给出了 TS-1 和 Silicalite-1 的紫外-可见漫反射光谱[23].TS-1 在 220 nm 有一个非常强的吸收谱带, 而不含 Ti 的 Silicalite-1 则没有这个吸收谱带. 220 nm 吸收谱带可以归属为 TS-1 中 O–Ti 之间的 p -d电荷跃迁. 图 3(b) 给出了 244 nm 激发光激发的 TS-1 的紫外共振拉曼光谱. 在 TS-1 和 Silicalite-1 拉曼光谱中都出现了 380 和 815 cm –1 的谱峰, 归属为分子筛骨架本身的特征. TS-1 分子筛中还出现了 3 个新的谱峰, 分别位于 490, 530 和 1 125 cm –1, 这 3 个谱峰来源于钛硅分子筛中骨架 Ti 物种, 归属为TS-1 分子筛中的骨架[Ti(OSi)4],本文中用 Ti–O–Si 指代这一结构. 1 125 cm −1 谱带归属为 Ti–O–Si 的非对称伸缩振动[24]. 理论计算表明, 1 125 cm −1谱峰归属为钛硅四面体[Ti(OSi)4]的全对称伸缩振动[9]. 在 325 和 488 nm 激发的 TS-1 分子筛的拉曼光谱中 (图 3(b)), 还有 3 个对应于锐钛矿相二氧化钛的拉曼谱峰, 分别位于 144, 390 和 637 cm –1, 说明该 TS-1 样品中还存在骨架外氧化钛物种. 然而, 这些谱峰在 244 nm 激发的拉曼光谱中并未出现. 这说明可200Raman shift (cm -1)Absorbance (a.u.)图 2 分子筛中骨架氧到过渡金属的荷电跃迁 (a)、紫外-可见光谱 (b) 和对应的共振拉曼光谱 (c)Fig. 2. Schematic of charge transfer transition between oxygen and transition metal ions in the framework of molecular sieves (a) and therelationship between UV-Vis spectra (b) and UV resonance Raman spectra (c). The Raman bands of X and Y components can be selectively enhanced by shifting the excitation laser lines towards their UV-Vis absorbance bands.Raman shift (cm -1)图 3 TS-1 和 Silicalite-1 的紫外-可见漫反射光谱 (a) 和TS-1 在 244, 325 和 488 nm 激发的拉曼光谱以及 Sili-calite-1 在 244 nm 激发的拉曼光谱 (b)Fig. 3. UV-Vis diffuse reflectance spectra (a) and Raman spectra excited with different laser lines at 244, 325, and 488 nm for Silicalite-1 and TS-1 (b).[23]第8期 范峰滔 等: 催化材料的紫外拉曼光谱研究 721见拉曼光谱对骨架外钛物种如 TiO 2 非常灵敏, 而紫外拉曼光谱仅对骨架钛物种灵敏.图 4 给出了 MCM-41 和 Ti-MCM-41 在 244 nm 激发的拉曼光谱. 相对于 MCM-41 而言, Ti-MCM-41 的拉曼光谱中存在 482, 520, 和 1 100 cm –1 三个新峰. 根据 TS-1 分子筛的拉曼谱峰归属, 这三个新峰归属为 Ti-MCM-41 无定形墙壁中四配位的钛物种. 与 TS-1 分子筛相比, Ti-MCM-41 的拉曼光谱中 1 110 cm –1 特征峰的频率较低(TS-1 中位于 1 125 cm −1), 表明 Ti-MCM-41 和 TS-1 分子筛中钛的配位环境明显不同. 在 TS-1 分子筛中, 钛原子被紧密地固定在分子筛的刚性骨架中, 而在 Ti-MCM-41 分子筛中, 钛原子的配位环境相对宽松[25].图 5 比较了 TS-1,Ti-MCM-41 和 Ti/SiO 2 中不同配位环境的钛物种相应的紫外拉曼特征谱峰. 由图可见, Ti–O–Si 的振动频率随配位环境的改变而改变. 处于 TS-1 中的刚性四配位骨架钛的特征谱峰位于 1 125 cm –1, 而当钛物种位于更柔性的配位环境中时, 如在 SiO 2 上, 钛的特征拉曼谱峰频率红移至 1 085 cm –1. 因此, 这个谱峰可以用来评价分子筛体系中钛的配位环境.1.2 Fe-ZSM-5 和 Fe-SBA-15 中孤立铁物种的鉴定用其它元素 (通常是过渡金属元素) 同晶取代微孔分子筛和介孔分子筛中的硅元素和铝元素是一种 非常有效的改变分子筛材料物理化学性质的方法[3].在分子筛骨架中, 即使存在痕量的三价元素, 也会明显改变分子筛的酸性以及相关的催化活性[27,28]. 铁离子交换的微孔分子筛如 Fe-ZSM-5 已经成功应用于许多催化过程[29~33],对 ZSM-5 骨架中铁的表征显得尤为重要. 而利用共振拉曼原理, 紫外拉曼光谱技术可以将 Fe-ZSM-5 分子筛中极低含量的骨架 Fe 可靠地鉴定出来.图 6(a) 给出了 Fe-ZSM-5 的紫外-可见漫反射光谱. 从图中可以看到有两个较强的吸收谱带分别位于 235 和 263 nm, 可归属为 Fe-ZSM-5 骨架中氧到铁Raman shift (cm -1)50010002507501250图 4 244 nm 激发的 MCM-41 和 Ti-MCM-41 (Si/Ti = 200)的紫外拉曼光谱图Fig. 4. UV Raman spectra of MCM-41 and Ti-MCM-41 (Si/Ti = 200) excited with 244 nm laser line.[25]图 5 TS-1, Ti-MCM-41 和 Ti/SiO 2 拉曼特征峰频率以及不同配位环境中钛物种的示意图Fig. 5. Characteristic Raman frequencies in UV Raman spectra of TS-1 (a), Ti-MCM-41 (b), and Ti/SiO 2 (c) and the schematic description of thecoordination environments of the titanium ions in the three materials.[26]722 催 化 学 报 第30卷的 p π-d π电荷跃迁. 图 6(b) 给出了 244, 325 和 532 nm 激发的 Fe-ZSM-5 的紫外拉曼光谱. 244 nm 激发线接近 Fe-ZSM-5 的 245nm 吸收谱带. 图中位于 290, 378, 460 和 800 cm −1 的谱峰是 MFI 结构的分子筛本身的信号[34],除此之外还出现了几个新谱峰, 分别位于 516, 1 016, 1 115 和 1 165 cm –1. 位于 516 和 1 115 cm –1 的谱峰可归属为骨架 Fe–O–Si 的对称和反对称伸缩振动. 1 016 cm –1 处谱峰源于骨架铁的引入导致的附近的 Si–O–Si 不对称伸缩振动[25,35].325 nm 激发的 Fe-ZSM-5 拉曼光谱中除了分子筛本身的信号外, 在 516, 1 115 和 1 165 cm −1 出现了较弱的拉曼谱峰, 在 1 016 cm –1 出现了较强的拉曼谱峰. 而当用 532 nm 激发时, Fe-ZSM-5 的拉曼光谱中除了分子筛本身信号外, 只有较强的 1 016 cm –1 谱峰 (图 6(b)).能被 244 nm 激发光激发的 1 165 cm –1谱峰是一个共振拉曼谱峰. 周期场密度泛函理论计算确认这个谱带来源于骨架中 FeO 4 物种的全对称伸缩振动[33]. 这一振动模式是由周围四个 Si–O–Si 物种的不对称伸缩振动驱动的. 可以说 1 165 cm –1 谱峰与分子筛骨架的晶化度有关, 这可以从结晶度较好的分子筛中该谱峰较强而得到验证[34].为了研究介孔分子筛材料 Fe-SBA-15 中骨架 Fe 物种的配位环境, 我们研究了 Fe-SBA-15 的紫外拉曼光谱. 图 7 给出了 244 和 325 nm 激发下的 Fe-SBA-15 的紫外拉曼光谱图. 在 244 nm 激发下, 除了 SBA-15 本身的 490, 600 和 800 cm –1 谱峰外,Fe-SBA-15 在 510 和 1 090 cm –1 处出现了两个新的谱峰, 可以归属为四配位 Fe–O–Si 的对称和反对称伸缩振动[36].325 nm 激发的 Fe-SBA-15 的拉曼谱图中仅能看到位于 978 cm –1 的谱峰, 244 nm 激发的 Fe-SBA-15 的拉曼谱图中也存在该峰, 它源于骨架铁引入导致的附近的 Si–O–Si 不对称伸缩振动[36].总之, 在 Fe-ZSM-5 拉曼谱图中共出现了四个谱峰, 分别位于 516, 1 016, 1 115 和 1 165 cm –1,而相应的 Fe-SBA-15 谱图中仅出现了 510, 978 和 1090 cm –1 三个谱峰, 说明在这两个体系中 Fe 的配位环境并不相同. Fe-ZSM-5 中刚性配位的四面体铁的拉曼谱峰位于 1 115 cm –1, 而在 Fe-SBA-15 中, 由于铁周围的配位环境更加柔性, 1 115 cm −1 谱峰位移至 1 090 cm –1. 在 TS-1 和 Ti-MCM-41 中也观察到了类似的趋势. 这一规律对于评价微孔材料和介孔材料中过渡金属物5001000378290800532325244116511151016Wavelength (nm)Raman shift (cm -1)I n t e n s i t y516200300400500600(b)(a)I n t e n s i t y244 nm325 nm 532 nm图 6 Fe-ZSM-5 的紫外-可见漫反射吸收光谱(a) 和在 244,325 和 532 nm 激发的拉曼光谱 (b)Fig. 6. UV-Vis diffuse reflectance spectra of Fe-ZSM-5 (a) and Raman spectra excitated at 244, 325, and 532 nm (b).[33]Raman shift (cm -1)50010001500图 7 Fe-SBA-15 在 244 和 325 nm 激发的紫外共振拉曼光谱Fig. 7. UV resonance Raman spectra of Fe-SBA-15 excited with 244 and 325 nm.[36]第8期范峰滔等: 催化材料的紫外拉曼光谱研究 723种的配位环境尤其重要.1.3V-MCM-41中不同V物种的鉴定在V-MCM-41的紫外-可见漫反射光谱中可以观察到位于270,340,410和450nm处的弱的电子吸收带,而在Si-MCM-41的谱图中并没有观察到相应的电子吸收带[37].270和340nm的吸收带可归属为骨架中四面体氧配体和中心V5+离子之间的荷电跃迁[38].图8是244nm激发得到的Si-MCM-41和V-MCM-41的紫外拉曼光谱.在V-MCM-41的紫外拉曼光谱中观察到930和1 070cm–1两个新谱峰. 930cm–1的谱峰可以归属于半骨架位的聚合态钒氧物种的V=O对称伸缩振动[38].1 070cm–1的谱峰可以归属于孤立形态钒氧物种的V=O对称伸缩振动[38].由于244nm的激发线接近V-MCM-41的电子吸收带,因此位于930和1 070cm–1的两个谱峰由于共振拉曼效应而得到增强.V-MCM-41中骨架V物种处于扭曲的四面体形态,这种结构可能具有较强的结构张力.实验结果证实,这种结构实际上是一种介稳态的结构,它很容易在较高的温度下聚合成聚集态的物种,当升高温度焙烧V-MCM-41时,930 cm–1处的谱带增强,而1 070cm−1处的谱带减弱.1 070和930cm–1处的谱带强度分别代表了表面孤立(骨架)和聚集态(骨架外)的钒物种的浓度.降低Si/V比或增加V的含量,与孤立钒物种相关的谱峰1 070cm–1稍减弱,而聚集态钒物种的谱峰930cm–1得到增强.进入V-MCM-41骨架中的V的量似乎是有限的,当V物种的量超过一定限度时,聚集态的钒氧物种(骨架外)将随钒物种含量的增加而增加.2微孔材料晶化机理的紫外拉曼光谱研究微孔和介孔材料的合成机理是材料合成科学中一个非常重要的课题.尽管到目前为止大量的分子筛结构已经被成功地合成出来,但研究分子筛的合成机理对合理设计分子筛结构以及定向合成具有特殊结构和性质的分子筛仍具有非常重要的意义[39].尽管许多工作研究了分子筛的制备并提出了一些关于成核以及晶体生长的机理[40~42],甚至包括从小的初级结构单元的形成和消耗直至大的晶体的形成过程的描述[43,44],然而由于水热晶化过程的复杂性,分子筛的形成过程以及详细机理的研究仍然极具挑战性.很多技术如X射线衍射和散射[45]、核磁共振[46]以及电镜[47]等都被用来研究分子筛的合成机理,但许多研究都是在非原位状态下进行的,即定时从反应混合物中取出一部分,在淬灭反应后分析样品.然而,微孔分子筛材料是在相对较高的温度和压力的水热条件下合成的,常规表征所必需的样品淬灭等步骤可能导致中间物种的结构变化[48],而且有机模板剂和无机物种之间一些弱的相互作用也会受到严重影响.原位技术是表征分子筛合成机理非常有效的手段,但是对于原位技术的研究开发相对较少[49].原位表征可以直接探测分子筛形成过程中的中间物种而不需要淬灭反应.原位技术不但可以连续检测反应,而且可以研究真实条件下的反应.因此,发展原位表征方法对在实际合成条件下研究分子筛的合成机理有着非常重要的意义.由于水的拉曼散射截面很小,所以拉曼光谱是一种非常适合于研究分子筛合成体系固相和液相的技术[49~51].紫外拉曼光谱可以有效地避免来自水热条件下生成的羟基化合物以及模板剂所产生的荧光,是表征分子筛结构的有力手段.这些优点使得紫外拉曼光谱成为一种可以原位研究分子筛合成机理的潜在工具[52].同时,利用共振拉曼效应可以灵敏地、选择性地检测骨架的过渡金属离子,这使紫外拉曼光谱特别适合研究过渡金属取代的分子筛的合成机理.图8Si-MCM-41和不同Si/V比的 V-MCM-41的紫外共振拉曼光谱图及V-MCM-41中两种V物种结构示意图Fig. 8. UV resonance Raman spectra of Si-MCM-41 and V-MCM-41 with different Si/V ratios (a) and schematic description of the two vanadium species incorporated in MCM-41 (b).[38]724 催 化 学 报 第30卷2.1 Fe-ZSM-5 合成机理的紫外拉曼光谱研究前面的工作已经提到, 利用不同激发线 (244 和 325 nm) 的紫外拉曼光谱可以选择性地得到骨架结构和铁物种的配位环境. 利用 325 nm 作为激发线的紫外拉曼光谱可以用来监测分子筛骨架的形成, 而利用 244 nm 作为激发线的紫外拉曼光谱可以选择性得到分子筛中有关铁的配位信息.图 9 是不同晶化时间的 Fe-ZSM-5 的紫外共振拉曼光谱. 在 325 nm 激发时 (图 9(a)), 前驱体有两个特征拉曼谱峰, 分别位于 460 和 984 cm −1. 460 cm −1 处的谱峰可以在无定形二氧化硅或玻璃状石英的拉曼谱图上观察到[53],归属于五元环和六元环的 Si–O–Si 的对称伸缩振动. 980 cm −1 附近的谱峰通常与骨架铁物种附近的 Si–O–Si 有关[25,54]. 随着反应的进行, 位于 460 和 984 cm −1 处的谱峰逐渐变得突出, 而后者的频率移到了更高的波数. 同时, 在 378 cm −1 处出现了一个肩峰, 表明在无定形凝胶中形成了少量类似于 MFI 结构的物种. 另外, 位于 460 cm −1 处的谱峰强度增加, 说明无定形凝胶中的五元环和六元环数目随着水热合成的进行而增加. 这表明在合成初期, 前驱体中的硅铝物种互相连接形成了五元和六员环这样的次级结构单元.随着晶化时间的进一步延长 (24 h), 合成物的拉曼光谱中出现了对应于 MFI 结构的特征谱峰 (290,380, 460 和 800 cm −1), 同时中心波数在 460 cm −1 处的相对弱的宽峰依然存在. 这表明无定形体系中形成了大量的具有 MFI 结构的晶体. 此外, 前驱体在 984 cm −1 处的拉曼谱峰在整个合成过程中逐渐移到了更高的波数, 晶化 36 h 后位移到了 1 016 cm −1 处, 表明在四面体配位铁物种附近的 Si–O–Si 结构变得刚性化[34].在 244 nm 下激发时 (图 9(b)), 随着水热合成时间的延长, 524 cm −1 处的宽峰变得强而尖锐, 而且移至低频 516 cm −1. 与此同时, 984 和 1 060 cm −1 处的谱峰分别蓝移至 1 016 和 1 115 cm −1. 这表明在 Fe-ZSM-5 形成的初期已经存在大量四面体配位的Fe-(OSi)4,然而它们的配位环境不如在晶化完全的分子筛骨架中那样刚性. 晶化初期处于扭曲四面体配位的铁原子在晶化过程中经历了显著的变化, 最后转变成了晶型 Fe-ZSM-5 分子筛骨架中均一且刚性的配位状态. 晶化 6 h 后的样品在 1 165 cm −1 处出现了一个肩峰, 而后该谱峰随着晶化时间的增加逐渐变强、变窄. 在晶化超过 36 h 后, 该谱峰变得特别突出. 研究表明, 该谱峰与分子筛骨架的结晶度有关[33,34].这一归属被晶化完全的具有 MFI 结构的样品在 1 165 cm −1处具有强而显著的谱峰所证实. 值得注意的是, 以 325 nm 为激发线的拉曼谱图中 984 cm −1 处的谱峰与244 nm为激发线的这一谱峰图 9 不同晶化时间的 Fe-ZSM-5 (Si/Fe=152)在 325 nm (a) 和 244 nm (b) 激发的紫外拉曼光谱以及 Fe-ZSM-5 的晶化机理 (c)Fig. 9. UV resonance Raman spectra of Fe-ZSM-5 (Si/Fe = 152) with different crystallization time excited with 325 nm (a) and 244 nm (b) and a proposed scheme for the formation mechanism of Fe-ZSM-5 (c).[34]第8期范峰滔等: 催化材料的紫外拉曼光谱研究 725明显不同.前驱体中325nm作为激发线的拉曼光谱中的984cm−1处的谱峰,随着晶化时间的延长逐渐且连续地移到了更高的频率,最后在晶化36h后出现在1 016cm−1处.而244nm为激发线的拉曼光谱中,984cm−1处的谱峰在晶化24h内频率基本保持不变,而在晶化30h后突然移至1 008cm−1,最后在晶化完全的Fe-ZSM-5中该谱峰移至1 016cm−1.244nm为激发线时的984cm−1拉曼谱峰的变化明显滞后于325nm为激发线的相应谱峰.同时,MFI结构的特征拉曼谱带(380和800cm−1)在244nm(图9(b),30h)为激发线时出现的时间也比325nm(图9(a),18h)为激发线时晚.这种滞后现象是由于样品对不同激发线(244和325nm)的吸收能力不同引起的[55].拉曼光谱结果明确地表明,Fe-ZSM-5骨架的形成是从样品核心开始的,然后由内到外逐渐晶化,这也被高分辨透射电镜结果所证实[34].图9(c)给出了一个Fe-ZSM-5形成的可能机理, Fe-ZSM-5的晶化是由合成初级阶段形成的扭曲四面体配位的Fe-(OSi)4物种和五元环、六元环硅物种通过聚集、成核而形成的.成核发生在无定形相的中心,无定形相中心晶化的Fe-ZSM-5通过消耗无定形壳层处于扭曲四面体配位的铁物种和硅物种而形成大的晶体,最终形成晶化完全的样品.2.2原位紫外拉曼光谱研究X分子筛的合成机理为了更好地理解水热合成,我们设计了用于水热合成分子筛的拉曼原位池[56],以模拟高温高压下反应斧中合成反应的真实情况.原位池被铜制的加热线圈所环绕.将一个透镜用硅橡胶密封于原位池的顶部,用来将激光聚焦到原位池中被研究样品的表面上.利用透镜作为原位池的窗口所获得的拉曼信号是利用平面镜作为窗口获得的拉曼信号的3~4倍.在分子筛合成的水热条件下,它能够经受住523 K的高温和4MPa的高压.为了研究合成反应中的液相和固相,实验中采用了两种类型的样品池:一个是深的样品池,它可使激光聚焦到液相中,用来研究合成中液相的变化;另一个是浅的样品池,它可使激光聚焦于液相和固相的分界面上,用来研究合成中固相的变化(见图10).我们利用原位紫外拉曼光谱研究了水热合成条件下X分子筛的固相和液相中物种的变化.在液相最初的拉曼光谱中有774和920cm−1[56]两个谱带,归属为液相中的单态硅物种[57~59].774cm−1谱峰的强度随着晶化时间的增加而逐渐加强,晶化到120min 时达到最大值,随后随着反应时间的延长强度逐渐下降.这说明合成过程中存在大量的单态硅物种,它们参与了整个晶化过程.该过程可以描述如下:在最初阶段,无定形硅凝胶和液相中的物种处于溶解平衡状态,随着反应时间的延长,大量的单态硅物种从硅凝胶前体中解聚出来;随着反应的进行,晶体的生长需要更多的单态硅物种;最后所有无定形前体被消耗并转变为晶型结构,不再解聚出单态硅物种.图11(a)给出了在373K水热条件下固相变化的原位拉曼光谱图.图中比较尖的500cm−1谱峰的存在说明前体中含有大量的四元环结构单元[60,61].随着反应时间的增加,该谱峰变得更加突出并且位移到514 cm−1,该峰归属于晶化完全的X型分子筛的四元环呼吸振动谱峰.随着反应的进行,对应于X型分子筛的特征峰298和380cm−1逐渐显现并且增强.这是由分子筛结构中双六元环的呼吸振动引起的.它们的出现和514cm−1谱峰的存在说明X型分子筛的形成是与四、六元环结构的存在密切相关的[35].图11(b)给出了514cm−1谱峰的强度随反应时间的变化曲线.晶化150min后,514cm−1处谱峰强度发生了明显的变化.值得注意的是,575cm–1谱峰在反应一开始就存在.它与硅铝前体相关并且随着反应的进行强度逐渐下降(图11(b)),一旦谱图中出现298和380cm–1谱峰,则575cm–1谱峰基本消失,说明575cm–1的谱峰图 10用于研究分子筛合成机理的原位拉曼样品池. (a)用于研究合成中液相组分变化的原位池内衬;(b)用于研究合成中固相组分变化的原位池内衬Fig.10.Schematic diagram of in situ Raman cells for hydrothermal synthesis of zeolites.(a) The sample holder inserted into the in situ Raman cell for the liquid phase study and the focus point; (b) The sample holder inserted into the in situ Raman cell for the solid phase study and the focus point.[56]。
二氧化钛光催化英文论文

Proceedings of the World Congress on Engineering and Computer Science 2010 Vol II WCECS 2010, October 20-22, 2010, San Francisco, USASynthesized Oxygen-Vacant TiO2 Nanopowders with Thermal Plasma Torch Evaporation Condensation ProcessC.-Y. Tsai, H.-C. Hsi and K.-S. Fanprecursors were required for processing. Theoretically, TiO2 nanoparticles with a high purity can be obtained by a direct combination of titanium atom and oxygen. The high melting point of titanium metal (i.e., 1941 K) makes the direct combination difficult to process. The sufficient energy provided by thermal plasma can vaporize metals with high melting points and makes the direct combination process possible [12]. Oxygen-deficient TiO2 (TiO2-X) has been extensively studied due to its photocatalytic properties under a visible light (VL) environment. Nakamura et al. prepared TiO2-X for NO removal. A ST-01 sample (Ishihara Sangyo Kaisha, 100% anatase) was treated with radio-frequency discharge using a reducing gas (H2) as the plasma gas. The authors concluded that the appearance of the VL activity was attributed to the newly formed oxygen vacancy state between the valence and the conduction bands in the TiO2 band structure [13]. Ihara et al. manufactured TiO2-X by calcinating the hydrolysis products of Ti(SO4)2 with NH3 in an ordinary electric furnace in dry air at 400 °C [14]. TiO2-X was generally developed by further modifying available TiO2 via reduction reactions or thermal treatments, thus a multiple step synthetic process was needed. Few studies were shown to produce TiO2-X in a single step. This study aimed at preparing TiO2-X nanopowders by direct combination of vaporized Ti atoms with oxygen. A single-step evaporation condensation was established using a transferred DC plasma torch as the heating source. The mixture of argon (Ar) and helium (He) was used as the plasma gas to achieve a high-temperature environment [15]. We considered this single-step process a potential method to produce high-quality nanoscale TiO2-X with clean surface and a narrow particle size distribution.Abstract—The objective of this research is to establish a novel process for preparing the oxygen-vacant titanium dioxide (TiO2-X) nanoparticles with a clean surface, high purity, and controllable size and dimension. Samples were synthesized via the gas-phase evaporation condensation method with transferred DC plasma torch as the heating source. TiO2-X nanopowders were fabricated using different ratios of Ar/He as the plasma gases. The formed TiO2-X nanoparticle was characterized with TEM, EDS and XRD. Experimental results showed that increasing the He addition to Ar plasma gas elevated the plasma temperature. High plasma temperature can cause the tungsten cathode to melt, gasify, and dope into TiO2 structure, which inhibited the size growth and the crystallinity of formed anatase. TiO2-X nanoparticles with anatase phase and particle size at 5−10 nm can be developed at the Ar/He = 75/25. The oxygen vacancy turned the color of TiO2 from white to yellow. Index Terms—oxygen vacancy, titanium dioxide, thermal, transferred DC plasma torch.I. INTRODUCTION Titanium dioxide (TiO2) has been used in numerous applications as a photocatalyst. The manufacturing process of TiO2 strongly influences the purity and physical properties of resulting nanoparticles; these properties subsequently affect the characteristics of TiO2 photocatalytic reactions. TiO2 nanoparticles can be synthesized via solution and vapor synthesis methods, such as sol-gel [1]–[3], thermal hydrolysis [4], hydrothermal processing [5], chemical vapor deposition [6] and evaporation condensation [7], [8]. Evaporation condensation has shown to possess advantages to develop nanoparticles and film with clean surface and a narrow particle size distribution. The heating sources for evaporation condensation include electron beam, laser and plasma [8]–[10]. Plasma has been referred to as an economic heating source and can provide sufficient energy to directly vaporize TiO2 precursors. Cold plasma such as microwave [11] was successfully used to prepare TiO2 photocatalyst. However, because the temperature of cold plasma was generally around room temperature, gaseousC.-Y. Tsai is with the Department of Safety, Health and Environment Engineering, National Kaohsiung First University of Science and Technology, 1, University Rd., Kaohsiung 824, Taiwan (e-mail: u9315918@.tw) H.-C. Hsi is with the Institute of Environmental Engineering and Management, National Taipei University of Technology, 1, Sec. 3, Chung-hsiao E. Rd., Taipei 106, Taiwan (corresponding author; phone: +886 -2-27712171ext4126; fax: +886-2-87732954; e-mail: hchsi@.tw) K.-S. Fan is with the Department of Safety, Health and Environment Engineering, National Kaohsiung First University of Science and Technology, 1, University Rd., Kaohsiung 824, Taiwan (e-mail: ksfan@.tw)II. EXPERIMENTAL Fig. 1 shows the schema of experimental apparatus for preparing TiO2-X nanoparticles using a thermal plasma torch. The system comprised a DC transferred plasma torch connected to a 5 kW DC power supply (Model PLA-250 A, Taiwan Plasma Corp., Taiwan), a water-cooled stainless steel reaction chamber, a stainless steel powder filter (Tee-Type Filters, Swagelok) and a vacuum pump for shifting the particle floated in the exhaust gas. The plasma torch consisted of a tungsten cathode, which was cooled in the central tunnel with water and in the suburbs with sheath gases (O2). Titanium metal with 99.8% purity was used as the anode; TiO2-X nanopowder was formed by the direct combination of evaporated titanium atoms from the anode target with oxygen gas (99.99% purity) within the thermal plasma environment. TiO2-X was synthesized at variousWCECS 2010ISBN: 978-988-18210-0-3 ISSN: 2078-0958 (Print); ISSN: 2078-0966 (Online)Proceedings of the World Congress on Engineering and Computer Science 2010 Vol II WCECS 2010, October 20-22, 2010, San Francisco, USAHe/Ar volume ratio (0/100, 15/85, 25/75, and 50/50) as plasma gas (total flow rate of 2 L/min; from position A in Fig. 1), the operating current of 110 A has been evaluated to be the optimal applied current for nanopowder synthesis. O2 was used as both the reacting gas and the shelter gas of plasma torch (2 L/min; from position B in Fig. 1). The tail gas containing the formed TiO2-X nanoparticles was passed through a stainless steel powder filter and a buffer tank induced by a vacuum pump.Reaction & Sheath gas Plasma gas Waternanoparticles at high plasma temperature. A more detailed discussion will be presented later in this paper. (a) (b)(c)(d)BAABTiTiO2Fig. 1. Schematic diagram of transferred DC plasma torch for producing oxygen-vacant TiO2 nanoparticles. The formed TiO2-X nanoparticles were collected using DI water from the stainless steel powder filter for sample characterization. A drop of suspension was dispersed in ethanol, placed onto a carbon-coated Cu grid followed by air-drying to remove the solvent, and then analyzed with a transmission electron microscope (TEM, Philips CM-200) to investigate samples’ morphology and particle size. The purity of nanoparticles was identified using an energy dispersive X-ray analyzer (EDS, OXFORD, JEOL JEM-2100F) operated at 200 kV accelerating voltage. Nanoparticles which placed on silver membrane filter paper. Powder X-ray diffraction (XRD, Shimadzu XRD-6000) was used for crystal structure identification. The scan range 2θ was between 20 and 80o with a scanning rate of 0.02o/s. III. RESULTS AND DISCUSSION Fig. 2 shows the TEM images of nanopowders synthesized at a fixed current of 110 A with various ratios of He/Ar as the plasma gas. It was observed that the diameter of the nanoparticles was 5−10 nm with 100% Ar as the plasma gas. When the He/Ar ratio increased to 15/85, the size of formed nanopowders appeared to increase to 5−50 nm. The size of nanoparticles grew at a larger He/Ar ratio is attributed to the greater thermal conductivity of He (0.144 at 273 K) as compared to that of Ar (0.0173 at 273 K). Therefore, the plasma formed at He/Ar = 15/85 possessed a higher temperature that caused the growth of TiO2 nuclei. If this proposed mechanism hold, TiO2 nanoparticles with a much larger size should be observed as the He/Ar mixing ratio greater than 15/85. The particle sizes of formed TiO2 at He/Ar = 25/75 and 50/50, however, were approximately 5−10 nm, indicating that the expected growth of TiO2 nuclei at an evaluated He/Ar ratio greater than 15/85 was not observed. The observed phenomena may be resulted by an unwanted tungsten contamination in the formed TiO2Fig. 2. TEM images of TiO2-X powders synthesized at a 110 A plasma current and He/Ar ratio of (a) 0/100, (b) 15/85, (c) 25/75 and (d) 50/50. (a)(b)Fig. 3. EDS images of TiO2 powders synthesized a 110 A plasma current and He/Ar ratio of (a) 0/100 and (b) 50/50. The EDS results for TiO2 prepared at a current of 110 A and 100/0 and 50/50 (Ar/He) plasma gases are shown in Fig. 3. The acquired results indicated that TiO2 nanopowders with a very high degree of purity were developed under the 100/0 plasma gas condition. A small amount of W was observed to melt and subsequently gasify from the plasma cathode and doped into the TiO2 nanopowders when 50% He was added to the plasma gas. Accompanying with the results obtained from the TEM analyses, it appeared that the gasified W doped into the formed TiO2 may restrain particles growth [16]–[18]. Lee et al. prepared nano-sized Al-doped TiO2 powders using a DC plasma jet as the heating source andWCECS 2010ISBN: 978-988-18210-0-3 ISSN: 2078-0958 (Print); ISSN: 2078-0966 (Online)Proceedings of the World Congress on Engineering and Computer Science 2010 Vol II WCECS 2010, October 20-22, 2010, San Francisco, USATiCl4 and AlCl3 as precursors. SEM results indicated that the size of particles decreased as increasing the amount of Al dopant [18]. The size reduction was attributed to the suppression of particle growth by Al species introduced into TiO2 crystal [19]. This consequence can interpret the doped metal restraining the nanoparticles growth, which is consonant with the upshot of TEM shown in Fig. 2.Ag A Ag Ag Ag A: anatase (d) Intensity (a.u.) A A(c)(b)temperature, which is elevating by adding 25% He into the Ar plasma gas. Notably, the anatase peaks for the sample synthesized at the He/Ar ratio of 50/50 are less sharp as compared to those obtained at the He/Ar ratio of 25/75. It was suspected that the melted W doped into the formed TiO2-X, causing defects in the crystal structure of anatase. A number of literatures [13], [14], [20], [22] presented that the TiO2 powder color changed from white to yellow due to oxygen vacancy in the crystal lattice of TiO2. The photographs of TiO2 nanopowders synthesized in the thermal plasma system showed that the color turned from white to yellow as increasing the He/Ar ratio (Fig. 5). Because the addition of He to Ar plasma gas elevated the plasma jet temperature, these observed results confirmed again that increasing the reaction temperature resulted in the oxygen vacancy of TiO2. IV. CONCLUSION(a)203040506070802 ThetaFig. 4. XRD patterns of the nanopowders synthesized at the He/Ar ratio of (a) 0/100, (b) 15/85, (c) 25/75 and (d) 50/50. (a) (b)TiO2-X nanoparticles was synthesized using a gas-phase evaporation condensation method with transferred plasma torch as the heating source. Results showed that TiO2 nanopowders with a very high degree of purity can be developed using 100% Ar as the plasma gas. The characteristics of synthesized nanopowders markedly affected by the amount of He added to the plasma gas. When 15% He was added to Ar plasma gas, the nanoparticles size grew to 5−50 nm at a plasma current of 110 A. Oxygen-vacant TiO2 (i.e., TiO2-X) was formed as He was added to the Ar plasma gas, which increased the plasma temperature. The tungsten melted and gasified from the plasma cathode doped into the TiO2 nanopowders, which influenced the crystal structures and powder size of formed TiO2-X. XRD examination demonstrated that the single crystal of anatase was obtained at 110 A and 25% He/Ar ratio = 25/75. The oxygen vacancy in TiO2 caused the color turning from white to yellow. ACKNOWLEDGMENT This work was supported by National Council of Taiwan (NSC94-2622-E-327-003-CC3) and Taiwan Plasma Corp., Kaohsiung, Taiwan. REFERENCES[1] H. Yamashita, M. Harada, J. Misaka, M. Takeuchi, K. Ikeue, and M. Anpo, “Degradation of propanol diluted in water under visible light irradiation using metal ion-implanted titanium dioxide photocatalysts,” J. Photochem. Photobiol. (a), vol. 148, pp. 257-261, 2002. S. P. Qiu, and S. J. Kalita, “Synthesis, processing and characterization of nanocrystalline titanium dioxide,” Mater. Sci. Eng. (a), vol. 435, pp. 327-332, 2006. M. Kanna, and S. Wongnawa, “Mixed amorphous and nanocrystalline TiO2 powders prepared by sol-gel method: Characterization and photocatalytic study,” Mater. Chem. Phys., vol. 110, pp. 166-175, 2008. M. C. Hidalgo, and D. Bahnemann, “Highly photoactive supported TiO2 prepared by thermal hydrolysis of TiOSO4: Optimisation of the method and comparison with other synthetic routes,” Appl. Catal. (b), vol. 61, pp. 259-266, 2005. X. H. Xia, Y. Liang, Z. Wang, J. Fan, Y. S. Luo, and Z. J. Jia, “Synthesis and photocatalytic properties of TiO2 nanostructures,” Mater. Res. Bull., vol. 43, pp. 2187-2195, 2008.(c)(d)Fig. 5. Photographs of the nanopowders synthesized at the He/Ar ratio of (a) 0/100, (b) 15/85, (c) 25/75 and (d) 50/50. Fig. 4 shows the XRD patterns of the TiO2 nanopowders synthesized at different He/Ar ratios. Because the TiO2-X nanopowders were laid on a silver membrane filter paper for XRD examination, four significant diffractive peaks contributed by Ag were shown. The XRD results clearly indicated that anatase was formed in the thermal plasma system, based on the observation of diffraction angle at 2θ = 25.28°. These results also suggested that anatase TiO2 with the greatest integrity of crystalline was obtained at the He/Ar ratio of 25/75. This result is expected because amorphous TiO2 can transform into anatase or rutile as increasingISBN: 978-988-18210-0-3 ISSN: 2078-0958 (Print); ISSN: 2078-0966 (Online)[2] [3][4][5]WCECS 2010Proceedings of the World Congress on Engineering and Computer Science 2010 Vol II WCECS 2010, October 20-22, 2010, San Francisco, USA[6] [7] [8] X. W. Zhang, M. H. Zhou, and L. C. Lei, “Preparation of anatase TiO2 supported on alumina by different metal organic chemical vapor deposition methods,” Appl. Catal. (a),vol. 282, pp. 285-293, 2005. U. Backman, U. Tapper, and J. K. Jokiniemi, “An aerosol method to synthesize supported metal catalyst nanoparticles,” Synth. Met., vol. 142, pp. 169-176, 2004. T. Sakai, Y. Kuniyoshi, W. Aoki, S. Ezoe, T. Endo, and Y. Hoshi, “High-rate deposition of photocatalytic TiO2 films by oxygen plasma assist reactive evaporation method,” Thin Solid Films, vol. 516, pp. 5860-5863, 2008. Y. Suda, H. Kawasaki, T. Ueda, and T. Ohshima, “Preparation of high quality nitrogen doped TiO2 thin film as a photocatalyst using a pulsed laser deposition method,” Thin Solid Films, vol. 453-54, pp. 162-166, 2004. S. H. Wang, T. K. Chen, K. K. Rao, and M. S. Wong, “Nanocolumnar titania thin films uniquely incorporated with carbon for visible light photocatalysis,” Appl. Catal. (b), vol. 76, pp. 328-334, 2007. M. Addamo, M. Bellardita, D. Carriazo, A. Di Paola, S. Milioto, L. Palmisano, and V. Rives, “Inorganic gels as precursors of TiO2 photocatalysts prepared by low temperature microwave or thermal treatment,” Appl. Catal. (b), vol. 84, pp. 742-748, 2008. C. J. Liu, G. P. Vissokov, and B. W. L. Jang, “Catalyst preparation using plasma technologies,” Catal. Today, vol. 72, pp. 173-184, 2002. I. Nakamura, N. Negishi, S. Kutsuna, T. Ihara, S. Sugihara, and E. Takeuchi, “Role of oxygen vacancy in the plasma-treated TiO2 photocatalyst with visible light activity for NO removal,” J. Mol. Catal. (a), vol. 161, pp. 205-212, 2000. T. Ihara, M. Miyoshi, Y. Iriyama, O. Matsumoto, and S. Sugihara, “Visible-light-active titanium oxide photocatalyst realized by an oxygen-deficient structure and by nitrogen doping,” Appl. Catal. (b), vol. 42, pp. 403-409, 2003. A. M. Fudolig, H. Nogami, J.-I. Yagi, K. Mimura, and M. Isshiki, “Prediction of surface temperature on metal beads subjected to argon-hydrogen transferred arc plasma impingement,” ISIJ, vol. 37, pp. 623-629, 1997. K.-N. P. Kumar, K. Keizer, and A. J. Burggraar, “Textural evolution and phase transformation in titania membranes: Part 1.–Unsupported membranes,” J. Mater. Chem., vol. 3, pp. 1141-1149, 1993. B. Xia, H. Z. Huang, and Y. C. Xie, “Heat treatment on TiO2 nanoparticles prepared by vapor-phase hydrolysis,” Mater. Sci. Eng. (b), vol. 57, pp. 150-154, 1999. J. E. Lee, S. M. Oh, and D. W. Park, “Synthesis of nano-sized Al doped TiO2 powders using thermal plasma,” Thin Solid Films, vol. 457, pp. 230-234, 2004. M. L. Taylor, G. E. Morris, and R. S. Smart, “Influence of aluminum doping on titania pigment structural and dispersion properties,” J. Colloid Interface Sci, vol. 262, pp. 81-88, 2003. T. Sekiya, K. Ichimura, M. Igarashi, and S. Kurita, “Absorption spectra of anatase TiO2 single crystals heat-treated under oxygen atmosphere,” J. Phys. Chem. Solids, vol. 61, pp. 1237-1242, 2000. T. Sekiya, S. Ohta, S. Kamei, M. Hanakawa, and S. Kurita, “Raman spectroscopy and phase transition of anatase TiO2 under high pressure,” J. Phys. Chem. Solids, vol. 62, pp. 717-721, 2001. K. S. Rane, R. Mhalsiker, S. Yin, T. Sato, K. Cho, E. Dunbar, and P. Biswas, “Visible light-sensitive yellow TiO2-XNX and Fe-N co-doped Ti1-yFeyO2-XNX anatase photocatalysts,” J. Solid State Chem., vol. 179, pp. 3033-3044, 2006.[9][10] [11][12] [13][14][15][16] [17] [18] [19] [20] [21] [22]ISBN: 978-988-18210-0-3 ISSN: 2078-0958 (Print); ISSN: 2078-0966 (Online)WCECS 2010。
- 1、下载文档前请自行甄别文档内容的完整性,平台不提供额外的编辑、内容补充、找答案等附加服务。
- 2、"仅部分预览"的文档,不可在线预览部分如存在完整性等问题,可反馈申请退款(可完整预览的文档不适用该条件!)。
- 3、如文档侵犯您的权益,请联系客服反馈,我们会尽快为您处理(人工客服工作时间:9:00-18:30)。
附录UV Raman Spectroscopic Study on TiO2. I. Phase Transformation at theSurface and in theBulkJing Zhang, Meijun Li, Zhaochi Feng, Jun Chen, and Can Li*State Key Laboratory of Catalysis, Dalian Institute of Chemical Physics, Chinese Academy of Sciences,P. O. Box 110, Dalian 116023, ChinaRecei V ed: September 16, 2005; In Final Form: No V ember 4, 2005Phase transformation of TiO2 from anatase to rutile is studied by UV Raman spectroscopy excited by 325and 244 nm lasers, visible Raman spectroscopy excited by 532 nm laser, X-ray diffraction (XRD), andtransmission electron microscopy (TEM). UV Raman spectroscopy is found to be more sensitive to the surfaceregion of TiO2 than visible Raman spectroscopy and XRD because TiO2 strongly absorbs UV light. Theanatase phase is detected by UV Raman spectroscopy for the sample calcined at higher temperatures thanwhen it is detected by visible Raman spectroscopy and XRD. The inconsistency in the results from the abovethree techniques suggests that the anatase phase of TiO2 at the surface region can remain at relatively highercalcination temperatures than that in the bulk during the phase transformation. The TEM results show thatsmall particles agglomerate into big particles when the TiO2sample is calcined at elevated temperatures andthe agglomeration of the TiO2 particles is along with the phase transformation from anatase to rutile. It issuggested that the rutile phase starts to form at the interfaces between the anatase particles in the agglomeratedTiO2 particles; namely, the anatase phase in the inner region of the agglomerated TiO2 particles turns out tochange into the rutile phase more easily than that in the outer surface region of the agglomerated TiO2 particles.When the anatase particles of TiO2 are covered with highly dispersed La2O3, the phase transformation inboth the bulk and surface regions is significantly retarded, owing to avoiding direct contact of the anataseparticles and occupying the surface defect sites of the anatase particles by La2O3.1. IntroductionTitania (TiO2) has been widely studied because of its uniqueoptical and chemical properties in catalysis,[1]photocatalysis,[2]sensitivity to humidity and gas,[3,4] nonlinear optics,[5]photoluminescence,[6]and so on. The two main kinds of crystalline TiO2,anatase and rutile, exhibit different physical and chemicalproperties. It is well-known that the anatase phase is suitablefor catalysts and supports,[7]while the rutile phase is used foroptical and electronic purposes because of its high dielectricconstant and high refractive index.[8]It has been well demonstratedthat the crystalline phase of TiO2 plays a significant rolein catalytic reactions, especially photocatalysis.[9-11]Some studieshave claimed that the anatasephase was more active than therutile phase in photocatalysis.[9,10]Although at ambient pressure and temperature the rutile phaseis morethermodynamically stable than the anatase phase,[12]anatase is the common phase rather than rutile because anataseis kinetically stable in nanocrystalline TiO2at relatively lowtemperatures.[13] It is believed that the anatase phase transformsto the rutile phase over a wide range of temperatures.[14]Therefore, understanding and controlling of the crystalline phaseand the process of phase transformation of TiO2 are important,though they are difficult.Many studies[13-31]have been done to understand the processof the phase transformation of TiO2. Zhang et al.[15]proposedthat the mechanism of the anatase-rutile phase transformationwas temperature-dependent according to the kinetic data fromX-ray diffraction (XRD). On the basis of transmission andscanning electron microscopies, Gouma et al.[16] suggested thatrutile nuclei formed on the surface of coarser anatase particlesand the newly transformed rutile particles grew at the expenseof neighboring anatase particles. Penn et al.[17]suggested thatthe formation of rutile nuclei at twin interfaces of anataseparticles heated hydrothermally.Catalytic performance of TiO2 largely depends on the surfaceproperties, especially the surface phase, because catalyticreaction takes place on the surface. The surface phase of TiO2should be responsible for its photocatalytic activity because notonly the photoinduced reactions take place on the surface[32] butalso the photoexcited electrons and holes might migrate throughthe surface region. Therefore, the surface phase of TiO2, whichis exposed to the light source, should play a crucial role inphotocatalysis. However, the surface phase of TiO2, particularlyduring the phase transformation, has not been investigated. Thechallenging questions still remain: is the phase in the surfaceregion the same as that in the bulk region, or how does thephase in the surface region of TiO2 particle change during thephase transformation of its bulk? The difficulty in answeringthe above questions was mainly due to lacking suitabletechniques that can sensitively detect the surface phase of TiO2.UV Raman spectroscopy is found to be more sensitive tothe surface phase of a solid sample when the sample absorbsUV light.[33]We studied the phase transition of zirconia (ZrO2)from tetragonal phase to monoclinic phase by UV Ramanspectroscopy, visible Raman spectroscopy, and XRD.[33] Theseresults clearly indicated that the surface phase of ZrO2 is usuallydifferent from the bulk phase of ZrO2 and the phase transforma-tion of ZrO2 starts from its surface region and then graduallydevelops into its bulk when the ZrO2 with tetragonal phase iscalcined at elevated temperatures.These findings lead us to further investigate the phasetransformation in the surface region of TiO2 by UV Ramanspectroscopy as TiO2 also strongly absorbs UV light. In thisstudy, we compared the Raman spectra of TiO2 calcined atdifferent temperatures with excitation lines in the UV and visibleregions. XRD and transmission electron microscopy (TEM)were also recorded to understand the process of phase transformationof TiO2. It was found that the results of UV Ramanspectra are different from those of visible Raman spectra andXRD patterns. The anatase phase of TiO2 at the surface regioncan remain at relatively higher temperatures than that in thebulk at elevated calcination temperatures; namely, the anatasephase in the inner region of the agglomerated TiO2 particlesturns out to change into the rutile phase more easily than thatin the outer surface region of the agglomerated TiO2 particles.The literature[15,17,19] proposed the mechanism that phasetransformation of TiO2 might start at the interfaces of contactinganatase particles. If the anatase particles of TiO2 are separated,the phase transformation of TiO2 from anatase to rutile couldbe retarded or prohibited. Jing et al.[34]showed that La3+ did notenter the crystal lattices of TiO2 and was uniformly dispersedonto TiO2in the form of lanthana (La2O3) particles with smallsize. To verify the above assumption, this study also preparedthe anatase phase of TiO2 sample covered with La2O3 andcharacterized the above sample by visible Raman spectroscopyand UV Raman spectroscopy. The results of the two types ofRaman spectra are in agreement with each other and show thatthe TiO2 particle covered with La2O3 can retain its anatase phaseboth in the bulk and in the surface region even after calcinationat 900 °C.2. Experimental Section2.1. Catalyst Preparation.2.1.1. Preparation of TiO2. TiO2was prepared by precipitation method. To 100 mL of anhydrousethanol was added 20 mL of titanium(IV) n-butoxide(Ti(OBu)4). This solution was added to a mixture solution ofdeionized water and 100 mL of anhydrous ethanol. The molarratio of the water/Ti(OBu)4was 75. After the formed whiteprecipitate was stirred continuously for 24 h, it was filtered andwashed twice with deionized water and anhydrous ethanol.Finally, the sample was dried at 100 °C and calcined in air attemperatures from 200 to 800 °C for 4 h, and then cooled toroom temperature.2.1.2. Preparation of La2O3-Co V ered TiO2(La2O3/TiO2). Theabove TiO2powder calcined at 500 °C was used as a support.The critical La2O3 loading corresponding to monolayer coverageof La2O3 on the grain surface of TiO2 is 0.27 g/100 m2. [35,36] Onthe basis of the BET surface area of the TiO2 support (54.3m2/g), the monolayer dispersion capacity can also be expressedas 15 wt % La2O3 of the weight of TiO2. La2O3/TiO2 samples,containing different amounts of La2O3(0.5-6 wt %) wereprepared by a wet impregnation method. The support wasimpregnated with aqueous solution of various concentrationsof lanthanum nitrate (La(NO3)3·6H2O) andsubsequently stirredin a hot water bath until it was dried. After the sample waskept at 110 °C overnight, it was calcined at 900 °C in air for 4h. A TiO2 sample was prepared by calcining the TiO2supportat 900 °C for 4 h (denoted as TiO2-900) for comparison withthe La2O3/TiO2 sample. Pure La2O3was obtained by calciningLa(NO3)3·6H2O at 550 °C for 4 h.2.2. Characterization.2.2.1. UV Raman Spectroscopy. UVRaman spectra were measured at room temperature with a Jobin-Yvon T64000 triple-stage spectrograph with spectral resolutionof 2 cm-1. The laser line at 325 nm of a He-Cd laser wasused as an exciting source with an output of 25 mW. The powerof laser at the sample was about3.0 mW. The 244 nm linefrom a Coherent Innova 300 Fred laser was used as anotherexcitation source. The power of the 244 nm line at sample wasbelow 1.0 mW.2.2.2. Visible Raman Spectroscopy. Visible Raman spectrawere recorded at room temperature on a Jobin-Yvon U1000scanning double monochromator with the spectral resolutionof 4 cm-1. The line at 532 nm from a DPSS 532 Model 200532 nmsingle-frequency laser was used as the excitation source.2.2.3. X-ray Powder Diffraction (XRD), TEM, and Ultra V iolet-Visible Diffuse Reflectance Spectroscopy. XRD patterns wereobtained on a Rigaku MiniFlex diffractometer with a Cu KRradiation source. Diffraction patterns were collected from 20°to 80°at a speed of 5°/min. TEM was taken on a JEM-2011TEM for estimating particle size and morphology. UV-visdiffuse reflectance spectra were recorded on a JASCO V-550UV-vis spectrophotometer.2.2.4. Brunauer-Emmett-Teller (BET) Specific SurfaceArea. The BET surface area of the TiO2 support was measuredby nitrogen adsorption at 77 K using a Micromeritics ASAP2000 adsorption analyzer.3. Results3.1. Spectral Characteristics of Anatase and Rutile TiO2.The anatase and rutile phases of TiO2 can be sensitivelyidentified by Raman spectroscopy based on their Raman spectra.The anatase phase shows major Raman bands at 144, 197, 399,515, 519 (superimposed with the 515 cm-1 band), and 639cm-1.[37] These bands can be attributed to the six Raman-activemodes of anatase phase with the symmetries of Eg, Eg, B1g,A1g, B1g, and Eg, respectively.[37] The typical Raman bands dueto rutile phase appear at 143 (superimposed with the 144 cm-1band due to anatase phase), 235, 447, and 612 cm-1, whichcan be ascribed to the B1g, two-phonon scattering, Eg, and A1gmodes of rutile phase, respectively.38Additionally, the band at144 cm-1is the strongest one for the anatase phase and theband at 143 cm-1 is the weakest one for the rutile phase. PartsA and B, respectively, of Figure 1display the Raman spectra ofTiO2 calcined at 500 and 800 °C with excitation lines at 532,325, and 244 nm. Obviously, both visible Raman spectra andUV Raman spectra show that the TiO2 sample is in the anatasephase (Figure 1A) and rutile phase (Figure 1B).Figure 2 shows UV-vis diffuse reflectance spectra of theTiO2 sample calcined at 500 and 800 °C (the TiO2 sample is inthe anatase phase and rutile phase, respectively). For the anatasephase, the maximum absorption and the absorption band edgecan be estimated to be around 324 and 400 nm, respectively.The maximum absorption and the absorption band edge shiftto a little longer wavelength for the rutile phase.[39]By comparing the Raman spectra of the anatase (Figure 1A)or rutile phase (Figure 1B) excited by 532, 325, and 244 nmlines, it is found that the relative intensities of characteristicbands due to anatase or rutile phase in the high-frequency regionare different. For the anatase phase (Figure 1A), the band at638 cm-1 is the strongest one in the Raman spectrum with theexcitation line at 325 or 532 nm, while the band at 395 cm-1 isthe strongest one in the Raman spectrum with the excitationline at 244 nm.For the rutile phase (Figure 1B), the intensities of the bandsat 445 and 612 cm-1 are comparable in the visible Ramanspectrum. The intensity of the band at 612 cm-1 is strongerthan that of the band at 445 cm-1 in the Raman spectrum withthe excitation line at 325 nm, and the reverse is true for theRaman spectrum with the excitation line at 244 nm. In addition,for the rutile phase, a band at approximately 826 cm-1 appearsin the UV Raman spectra. Some investigations show that therutile phase of TiO2 exhibits a weak band at 826 cm-1 assignedto the B2g mode.[38,40]The fact that the relative intensities of the Raman bands ofanatase phase or rutile phase are different for UV Ramanspectroscopy and visible Raman spectroscopy are mainly dueto the UV resonance Raman effect because the laser lines at325 and 244 nm are in the electronic absorption region of TiO 2(Figure 2). There is no resonance Raman effect observed forthe TiO 2 sample excited by visible laser line, because the lineat 532 nm is outside the absorption region of TiO 2 (Figure 2).Therefore, for the anatase or rutile phase, the Raman spectroscopiccharacteristics in the visible Raman spectrum are differentfrom those in the UV Raman spectrum. When the UV laserline with different wavelengths is used as the excitation source,the resonance enhancement effect on the Raman bands ofanatase or rutile phase is different. For example, for the rutilephase (Figure 1B), the band at 612 cm -1 is easily resonanceenhanced when the excitation wavelength is 325 nm. Amongall the characteristic bands of the rutile phase, the extent ofresonance enhancement of 445 cm -1 is the strongest when the244 nm laser is used as the excitation source (Figure 1B).3.2. Semiquantitative Analysis of the Phase Compositionof TiO 2 by XRD and Raman Spectroscopy. The weightfraction of the rutile phase in the TiO 2 sample, W R, can beestimated from the XRD peak intensities using the followingformula:[41]]84.801[1)(rut ana A A W R +=where A ana and A rut represent the X-ray integrated intensities ofanatase (101) and rutile (110) diffraction peaks, respectively.To estimate the weight fraction of the rutile phase in the TiO 2sample by Raman spectroscopy, pure anatase phase and purerutile phase of the TiO 2 sample, which have been prepared bycalcination of TiO 2 powder at 500 and 800 °C for 4 h, weremechanically mixed at given weight ratio and ground carefullyto mix sufficiently. Figure 3A displays the visible Raman spectra of the mechanicalmixture with 1:1, 1:5, 1:10, 1:15, 5:1, and 10:1 ratios ofanatase phase to rutile phase. The relationship between the arearatios of the visible Raman band at 395 cm-1 for anatase phaseto the band at 445 cm -1 for rutile phase (A 395 cm -1/A 445 cm -1) andthe weight ratios of anatase phase to rutile phase (W A /W R ) isplotted in Figure 3B. It can be seen that a linear relationshipbetween the band area ratios and the weight ratios of anatasephase to rutile phase in the mixture is obtained. The rutilecontent in the Degussa P25, which usually consists of roughlyabout 80% anatase and 20% rutile phase,42 was estimated bythis plot. Our Raman result indicates that the rutile content inthe Degussa P25 is about 18.7%, which is close to the knownresult. Thus, the above linear relationship based on visibleRaman spectroscopy can be used to estimate the rutile contentin TiO 2.Figure 4A presents the UV Raman spectra of the mechanicalmixture with 1:1, 1:2, 1:4, 1:6, 1:10, and 1:15 ratios of anatasephase to rutile phase with the excitation line at 325 nm. Figure4B shows the plot of the area ratios of the UV Raman band at612 cm-1 for rutile phase to the band at 638 cm-1 for anatasephase (A 612 cm -1/A 638 cm -1) versus the weight ratios of rutile phaseto anatase phase (W R/W A). There is also a linear relationshipbetween the band area ratios and the weight ratios of rutile phaseto anatase phase.3.3. Phase Transformation of TiO 2 at Elevated CalcinationTemperatures.3.3.1. XRD Patterns and Visible Raman Spectraof TiO2Calcined at Different Temperatures. Figure 5 showsthe XRD patterns of TiO2calcined at different temperatures.The “A” and “R” in the figure denote the anatase and rutilephases, respectively. For the sample before calcination, diffractionpeaks due to the crystalline phase are not observed,suggesting that the sample is still in the amorphous phase. Whenthe sample was calcined at 200 °C, weak and broad peaks at2θ=25.5°, 37.9°, 48.2°, 53.8°, and 55.0° were observed. Thesepeaks represent the indices of (101), (004), (200), (105), and(211) planes of anatase phase, respectively.[43] These results suggest that some portions of the amorphous phase transforminto the anatase phase. The diffraction peaks due to anatasephase develop with increasing the temperature of calcination.When the calcination temperature was increased to 500 °C, thediffraction peaks due to anatase phase became narrow andintense in intensity. This indicates that the crystallinity of theanatase phase is further improved.[44]When the sample was calcined at 550 °C, weak peaks wereobserved at 2θ=27.6°, 36.1°, 41.2°, and 54.3°, whichcorrespond to the indices of (110), (101), (111), and (211) planesof rutile phase. [43]This indicates that the anatase phase starts totransform into the rutile phase at 550 °C. The diffraction peaksof anatase phase gradually diminish in intensity and thediffraction patterns of rutile phase become predominant withthe calcination temperatures from 580 to 700 °C. These resultsclearly show that the phase transformation from anatase to rutileprogressively proceeds at the elevated temperatures. The diffractionpeaks assigned to anatase phase disappear at 750 °C,indicating that the anatase phase completely changes into therutile phase. The diffraction peaks of rutile phase became quitestrong and sharp after the sample was calcined at 800 °C, owingto the high crystallinity of the rutile phase.Figure 6 displays the visible Raman spectra of TiO2 calcinedat different temperatures. For the sample before calcination, twobroad bands at about 430 and 605 cm-1are observed, indicatingthat the sample is in the amorphous phase.[19]For the samplecalcined at 200 °C, a Raman band at 143 cm-1 is observed andthe high-frequency region shows interference from the fluorescencebackground, which might come from organic species.After calcination at 300 °C, other characteristic bands of anatasephase appear at 195, 395, 515, and 638 cm-1, but some portionsof the sample may exist in the amorphous phase because thereis still a broad background in Figure 6.It was found that, when the sample was calcined at 400 °C,the fluorescence disappeared, possibly because the organicresidues were removed by the oxidation. The bands of anatasephase increased in intensity and decreased in line width whenthe sample was calcined at 500 °C. This result suggests thatthe crystallinity of the anatase phase is greatly improved,[18] whichis confirmed by XRD (Figure 5). The enlarged section of Figure6 shows the Raman spectrum of the high-frequency region ofthe sample calcined at 500 °C. Besides the bands at 395, 515,and 638 cm-1, two very weak bands at 320 and 796 cm-1areobserved. These two bands can be assigned to a two-phononscattering band and a first overtone of B1g at 396 cm-1,respectively. [37]It is noteworthy that a very weak band appearsat 445 cm-1 due to rutile phase for the sample calcined at 550°C. This indicates that the anatase phase starts to change intothe rutile phase at 550 °C. This result is in good agreementwith that of XRD patterns (Figure 5). The weightpercentageof the rutile phase in the samples calcined at different temperatureswas estimated by visible Raman spectroscopy and XRD(shown in Figure 7). As seen from Figure 7, the rutile contentestimated from visible Raman spectrum and XRD pattern ofthe sample calcined at 550 °C is 4.2% and 5.7%, respectively.It can be seen that the rutile contents estimated by visible Ramanspectroscopy and XRD are also in accordance with each other.When the sample was calcined at 580 °C, other twocharacteristic bands were observed at 235 and 612 cm-1 due toRaman-active modes of rutile phase. Figure 7 shows the rutilecontent is 13.6% and 10.9% based on the visible Ramanspectrum and XRD pattern of the sample calcined at 580 °C.The intensities of the bands of rutile phase (235, 445, and 612cm-1) increased steadily while those of the bands of anatasephase (195, 395, 515, and 638 cm-1) decreased when thecalcination temperatures were elevated from 600 to 680 °C(Figure 6). These results suggest that the TiO2 sample undergoesthe phase transformation from anatase to rutile gradually. Therutile content was estimated for the samples calcined from 600to 680 °C based on the visible Raman spectra. The results showthat the content of the rutile phase is increased from 33.1% to91.2% respectively for the samples calcined at 600 and 680 °C(Figure 7). The XRD results corresponding to the above twosamples indicate that the rutile content is changed from 32.9%to 90.7% (Figure 7). These results clearly show that the rutilecontent in the sample estimated by visible Raman spectroscopyis agreement with that estimated by XRD.The Raman spectrum of the sample calcined at 700 °C showsmainly the characteristic bands of rutile phase, but the very weakbands of anatase phase are still observed (Figure 6). When thesample was calcined at 750 °C, the bands of anatase phasedisappeared and only the bands due to rutile phase (143, 235,445, and 612 cm-1) were observed. These results indicate thatthe anatase phase completely transforms into the rutile phaseand are consistent with the results from XRD (Figure 5). Whenthe temperature was increased to 800 °C, the characteristic bandsdue to rutile phase increased in intensity further.Both the results of XRD and visible Raman spectra (Figures5 and 6) show that the anatase phase appears at around 200 °Cand perfect anatase phase is formed after calcination at temperaturesof 400-500 °C. The rutile phase starts to form at 550°C, and the anatase phase completely transforms into the rutilephase at 750 °C. The signals of visible Raman spectra comemainly from the bulk region of TiO2because the TiO2 sampleis transparent in the visible region (Figure 2).[33]XRD is knownas a bulk-sensitive method. Therefore, it is essentially inagreement between the results of visible Raman spectra andXRD patterns.3.3.2. UV Raman Spectra of TiO2 Calcined at DifferentTemperatures. UV-vis diffuse reflectance spectra (Figure 2)clearly show that TiO2 has strong electronic absorption in theUV region. Thus, the UV Raman spectra excited by a UV laserline contain more signal from the surface skin region than thebulk of the TiO2 sample because the signal from the bulk isattenuated sharply due to the strong absorption.[33] Therefore, ifa UV laser line in the absorption region of TiO2is used as theexcitation source of Raman spectroscopy, the information fromUV Raman spectra is often different fromthat of visible Ramanspectra.The laser line at 325 nm was selected as the excitation sourceof the UV Raman spectra. The UV Raman spectra and thecontent of the rutile phase of the TiO2 sample calcined atdifferent temperatures are shown in parts A and B, respectively,of Figure 8. When the sample was calcined at 200 or 300 °C,the Raman band at 143 cm-1 with a shoulder band at 195 cm-1and three broad bands at 395, 515, and 638 cm-1 were observed,indicating that the anatase phase is formed in the sample.However, the low intensity and the broad band indicate thatthe amorphous phase still remains in the sample. It can be seenthat the fluorescence in the high-frequency region can be avoidedwhen the UV laser line is used as the excitation line. However,the corresponding visible Raman spectra (Figure 6) showinterference from the fluorescence.All bands assigned to anatase phase become sharp and strongafter calcination at 500 °C (Figure 8A). These results are inagreement with those of XRD and visible Raman spectra(Figures 5 and 6). The UV Raman spectra of the sample withthe calcination temperatures from 550 to 680 °C are essentiallythe same as those of the sample calcined at 500 °C (Figure 8A).However, according to the XRD patterns and visible Ramanspectra (Figures 5 and 6), the anatase phase starts to transforminto the rutile phase at only 550 °C and the anatase phasegradually changes into the rutile phase in the temperature rangeof 550-680 °C.After calcination at 700 °C, a new band at 612 cm-1 andtwo weak bands at 235 and 445 cm-1 due to rutile phase appearwhile the intensities of the bands of anatase phase begin todecrease (Figure 8A). On the basis of the UV Raman spectrumand XRD pattern of the sample calcined at 700 °C, the rutilecontent in the sample is 56.1% and 97.0%, respectively (Figure8B). It is found that the rutile content estimated by UV Ramanspectroscopy is far less than that estimated by XRD.When the sample was calcined at 750 °C, the intensities ofthe bands due to rutile phase increased, but the intensities ofthe bands due to anatase phase were still strong in the UVRaman spectra (Figure 8A). The UV Raman spectrum of thesample calcined at 750 °C indicates that the rutile content is84.3% (Figure 8B). However, the results of XRD and visibleRaman spectrum (Figures 5 and 6) suggest that the anatase phasetotally transformed into the rutile phase after the sample wascalcined at 750 °C. The characteristic bands due to anatase phasedisappear and the sample is in the rutile phase after calcinationat 800 °C (Figure 8A). Obviously, there are distinct differences between the results from the UV Raman spectra, visible Ramanspectra, and XRD patterns. It seems that the anatase phaseremains at relatively higher temperatures when detected by UVRaman spectroscopy than by XRD and visible Raman spectroscopy. Another UV laser line at 244 nm was also selected as theexcitation source of UV Raman spectroscopy in order to getfurther insights into the phase transformation of TiO2. Theresults of the UV Raman spectra of TiO2 calcined at differenttemperatures with the excitation line at 244 nm are presentedin Figure 9. When the sample was calcined at 200 °C, four broadbands were observed at 143, 395, 515, and 638 cm-1, whichclearly indicate that the anatase phase exists in the sample. Theintensities of the Raman bands due to anatase phase (143, 395,515, and 638 cm-1) become strong after calcination at 500 °C.The UV Raman spectra hardly change for the sample calcinedatdifferent temperatures even up to 680 °C. The characteristicbands (445 and 612 cm-1) of rutile phase appear only whenthe calcination temperature exceeds 700 °C. This result is ingood agreement with that from the UV Raman spectrum of thesample calcined at 700 °C with 325 nm excitation (Figure 8A).The intensities of the bands due to anatase phase (395, 515,and 638 cm-1) decrease while those of bands assigned to rutilephase (445 and 612 cm-1) increase after calcination at 750 °C(Figure 9). When the sample was calcined at 800 °C, the Ramanbands due to anatase phase disappeared, while the bands of rutilephase developed. This result indicates that the sample calcinedat 800 °C is in the rutile phase. It is interesting to note that theresults of the UV Raman spectra with the excitation lines at325 and 244 nm are in agreement with each other but aredifferent from those of XRD patterns and visible Raman spectra.3.3.3. TEM of the TiO2 Sample Calcined at DifferentTemperatures. TEM was used to characterize the microstructureof the TiO2 sample calcined at 500, 600, and 800 °C (shown inFigure 10). Most particles in the sample calcined at 500 °Cexhibit diameters in a range between 10 and 30 nm (Figure10a). On the other hand, remarkable agglomeration is observedfor the TiO2 sample calcined at 500 °C. The particle sizeincreases after calcination at 600 °C (Figure 10b). Accordingto the results of XRD and visible Raman spectra (Figures 5and 6), the sample undergoes the phase transformation fromanatase to rutile gradually in the temperature range of 550-680 °C. These results imply that the phase transformation andgrowth of the particle size are interrelated. Many researchers[45-49]reported similar phenomena. Kumar et al.45 attributed thisparticle size growth to the higher atomic mobility because ofbond breakage during the phase transformation. When thecalcination temperature was increased to 800 °C, TiO2 particlesfurther grew and the particle size could be as large as about200 nm (Figure 10c).3.4. Visible Raman Spectra and UV Raman Spectra ofLa2O3/TiO2 with Increasing La2O3 Loading. The literature[15,17,29]proposed the mechanism that the phase transformationof TiO2might start at the interfaces of contacting anataseparticles. If direct contact between anatase particles of TiO2 isavoided, the phase transformation of TiO2 from anatase to rutilecould be retarded or prohibited. This assumption may be verifiedby covering the surface of anatase TiO2 with an additive. Inthis work, we used La2O3dispersed on anatase TiO2to preventthe anatase particles from contacting directly because it wasreported that La2O3could be highly dispersed on anataseTiO2.[34-36]Figure 11A displays the visible Raman spectra of La2O3/TiO2with increasing La2O3 loading. TiO2 support is in the anatasephase because only characteristic bands (143, 195, 395, 515,and 638 cm-1) due to anatase phase are observed. When theTiO2 support was calcined at 900 °C (TiO2-900), the Ramanspectrum gave the characteristic bands of rutile phase, indicatingthat the TiO2-900 sample was in the rutile phase. When thesample with 0.5 wt % La2O3 loading was calcined at 900 °C,the Raman spectrum drastically changed, as compared to theTiO2-900 sample. Only characteristic bands of anatase phasewere observed, suggesting that the TiO2 sample retains itsanatase phase when La2O3 loading is 0.5 wt % while the TiO2-900 sample is in the rutile phase. The。