SoilDection变量施肥系统
土力学英语

1.geotechnical engineering岩土工程2.foundation engineering基础工程3.soil, earth土4.soil mechanics土力学cyclic loading周期荷载unloading卸载reloading再加载viscoelastic foundation粘弹性地基viscous damping粘滞阻尼shear modulus剪切模量5.soil dynamics土动力学6.stress path应力路径7.numerical geotechanics 数值岩土力学二. 土的分类1.residual soil残积土 groundwater level地下水位2.groundwater 地下水 groundwater table地下水位3.clay minerals粘土矿物4.secondary minerals次生矿物ndslides滑坡6.bore hole columnar section钻孔柱状图7.engineering geologic investigation工程地质勘察8.boulder漂石9.cobble卵石11.gravelly sand砾砂12.coarse sand粗砂13.medium sand中砂14.fine sand细砂15.silty sand粉土16.clayey soil粘性土17.clay粘土18.silty clay粉质粘土19.silt粉土20.sandy silt砂质粉土21.clayey silt粘质粉土22.saturated soil饱和土23.unsaturated soil非饱和土24.fill (soil)填土25.overconsolidated soil超固结土26.normally consolidated soil正常固结土27.underconsolidated soil欠固结土28.zonal soil区域性土29.soft clay软粘土30.expansive (swelling) soil膨胀土31.peat泥炭32.loess黄土33.frozen soil冻土。
Similar response of labile and resistant soil organic matter pools to changes in temperature-nature

spectrometer equipped with a automatic carbonate preparation system(CAPS).Results are reported relative to the Vienna Pee Dee Belemnite standard(VPDB).Standard external analytical precision,based on replicate analysis of in-house standards calibrated to NBS-19,is better than0.1‰for d18O and d13C.Received1September;accepted25October2004;doi:10.1038/nature03135.1.Broecker,W.S.&Peng,T.-H.The role of CaCO3compensation in the glacial to interglacialatmospheric CO2change.Glob.Biogeochem.Cycles1,15–29(1987).2.Van Andel,T.H.Mesozoic/Cenozoic calcite compensation depth and the global distribution ofcalcareous sediments.Earth Planet.Sci.Lett.26,187–194(1975).3.Kennett,J.P.&Shackleton,N.J.Oxygen isotopic evidence for the development of the psychrosphere38Myr ago.Nature260,513–515(1976).ler,K.G.,Wright,J.D.&Fairbanks,R.G.Unlocking the ice house:Oligocene-Miocene oxygenisotopes,eustasy,and margin erosion.J.Geophys.Res.96,B4,6829–6849(1991).5.Zachos,J.C.,Quinn,T.M.&Salamy,K.A.High-resolution(104years)deep-sea foraminiferal stableisotope records of the Eocene-Oligocene climate transition.Palaeoceanography11,251–266(1996).6.Lear,C.H.,Elderfield,H.&Wilson,P.A.Cenozoic deep-sea temperatures and global ice volumesfrom Mg/Ca in benthic foraminiferal calcite.Science287,269–272(2000).7.DeConto,R.M.&Pollard,D.Rapid Cenozoic glaciation of Antarctica triggered by decliningatmospheric CO2.Nature421,245–249(2003).8.Shipboard Scientific Party2002.Leg199summary.Proc.ODP Init.Rep.(eds Lyle,M.W.,Wilson,P.A.&Janecek,T.R.)199,1–87(2002).skar,J.et al.Long term evolution and chaotic diffusion of the insolation quantities of Mars.Icarus170,343–364(2004).10.Peterson,L.C.Backman,te Cenozoic carbonate accumulation and the history of the carbonatecompensation depth in the western equatorial Indian Ocean.Proc.ODP Sci.Res.(eds Duncan,R.A., Backman,J.,Dunbar,R.B.&Peterson,L.C.)115,467–489(1990).11.Salamy,K.A.&Zachos,test Eocene-Early Oligocene climate change and Southern Oceanfertility:inferences from sediment accumulation and stable isotope data.Palaeogeogr.Palaeoclimatol.Palaeoecol.145,61–77(1999).12.Ehrmann,W.U.&Mackensen,A.Sedimentological evidence for the formation of an East Antarcticice sheet in Eocene/Oligocene time.Palaeogeogr.Palaeoclimatol.Palaeoecol.93,85–112(1992). 13.Ivany,L.C.,Patterson,W.P.&Lohmann,K.C.Cooler winters as a possible cause of mass extinction atthe Eocene/Oligocene boundary.Nature407,887–890(2000).14.Pa¨like,H.,Laskar,J.&Shackleton,N.J.Geologic constraints on the chaotic diffusion of the solarsystem.Geology32(11),929–932doi:10.1130/G20750(2004).15.Billups,K.&Schrag,D.P.Application of benthic foraminiferal Mg/Ca ratios to questions of Cenozoicclimate change.Earth Planet.Sci.Lett.209,181–195(2003).16.Lear,C.H.,Rosenthal,Y.,Coxall,H.K.&Wilson,te Eocene to early Miocene ice-sheetdynamics and the global carbon cycle.Paleoceanography19,doi:10.1029/2004PA001039(2004). 17.Pekar,S.F.,Christie-Blick,N.,Kominz,M.A.&Miller,K.G.Calibration between eustatic estimatesfrom backstripping and oxygen isotopic records for the Oligocene.Geology30,903–906(2002). 18.Lythe,M.B.,Vaughan,D.G.&BEDMAP Consortium,BEDMAP:A new ice thickness and subglacialtopographic model of Antarctica.J.Geophys.Res.106,B6,11335–11351(2001).19.Huybrechts,P.Sea-level changes at the LGM from ice-dynamic reconstructions of the Greenland andAntarctic ice sheets during the glacial cycles.Quat.Sci.Rev.21,203–231(2002).20.Hindmarsh,R.C.A.Time-scales and degrees of freedom operating in the evolution of continental ice-sheets.Trans.R.Soc.Edinb.Earth Sci.81,371–384(1990).21.Davies,R.,Cartwright,J.,Pike,J.&Line,C.Early Oligocene initiation of North Atlantic deep waterformation.Nature410,917–920(2001).22.Archer,D.&Maier-Reimer,E.Effect of deep-sea sedimentary calcite preservation on atmosphericCO2concentration.Nature367,260–263(1994).23.Sigman,D.M.&Boyle,E.A.Glacial/interglacial variations in atmospheric carbon dioxide.Nature407,859–869(2000).24.Zeebe,R.E.&Westbroek,P.A simple model for the CaCO3saturation state of the ocean:The“Strangelove,”the“Neritan,”and the“Cretan”Ocean.Geochem.Geophys.Geosyst.4,1104(2003).25.Kump,L.R.&Arthur,M.A.in Tectonics Uplift and Climate Change(ed.Ruddiman,W.F.)399–426(Plenum,New York,1997).26.Zachos,J.C.,Opdyke,B.N.,Quinn,T.M.,Jones,C.E.&Halliday,A.N.Early Cenozoic glaciation,Antarctic weathering and seawater87Sr/86Sr;is there a link?Chem.Geol.161,165–180(1999). 27.Ravizza,G.&Peucker-Ehrenbrink,B.The marine187Os/188Os record of the Eocene-Oligocenetransition:the interplay of weathering and glaciation.Earth Planet.Sci.Lett.210,151–165(2003).28.Berger,W.H.&Winterer,E.L.in Plate Stratigraphy and the Fluctuating Carbonate line in PelagicSediments:On Land and Under the Sea(eds Hsu¨,K.J.&Jenkyns,H.C.)11–48(Int.Assoc.Sedimentologists Spec.Publ.1,Blackwell Science,Oxford,1974).29.Opdyke,B.N.&Wilkinson,B.H.Surface area control of shallow cratonic to deep marine carbonateaccumulation.Paleoceanography3,685–703(1989).30.Harrison,K.G.Role of increased marine silica input on paleo-pCO2levels.Paleoceanography15,292–298(2000).Supplementary Information accompanies the paper on /nature. Acknowledgements We thank the Shipboard Party of Ocean Drilling Program Leg199for assistance at sea and M.Bolshaw,M.Cooper and H.Birch for laboratory assistance.This work was supported by a NERC UK ODP grant to P.A.W.,a Royal Commission for the Exhibition of1851 fellowship awarded to H.K.C.and by Swedish Research Council(VR)funding to H.P.We thank W.Broecker,R.Hindmarsh,S.D’Hondt,A.Merico,Y.Rosenthal,R.Rickaby,J.Shepherd and T.Tyrrell for discussions and comments on an earlier draft and L.Kump for a constructive review.Competing interests statement The authors declare that they have no competingfinancial interests.Correspondence and requests for materials should be addressed to P.A.W.(paw1@)............................................................... Similar response of labile and resistant soil organic matterpools to changes in temperature Changming Fang1,Pete Smith1,John B.Moncrieff2&Jo U.Smith11School of Biological Sciences,University of Aberdeen,Aberdeen AB243UU,UK 2Ecology and Resource Management,School of GeoSciences,The University of Edinburgh,Edinburgh EH93JU,UK ............................................................................................................................................................................. Our understanding of the relationship between the decompo-sition of soil organic matter(SOM)and soil temperature affects our predictions of the impact of climate change on soil-stored carbon1.One current opinion is that the decomposition of soil labile carbon is sensitive to temperature variation whereas resistant components are insensitive2–4.The resistant carbon or organic matter in mineral soil is then assumed to be unresponsive to global warming2,4.But the global pattern and magnitude of the predicted future soil carbon stock will mainly rely on the temperature sensitivity of these resistant carbon pools.To inves-tigate this sensitivity,we have incubated soils under changing temperature.Here we report that SOM decomposition or soil basal respiration rate was significantly affected by changes in SOM components associated with soil depth,sampling method and incubation time.Wefind,however,that the temperature sensitivity for SOM decomposition was not affected,suggesting that the temperature sensitivity for resistant organic matter pools does not differ significantly from that of labile pools,and that both types of SOM will therefore respond similarly to global warming.The temperature sensitivity of SOM decomposition,commonly referred to as Q10,is critical for modelling changes in soil C stock3–6. The assumption that the decomposition of old organic matter2–3or organic C in mineral soil4does not vary with temperature—that is, that the decomposition of labile C pools are sensitive,but resistant pools are insensitive,to temperature perturbations—suggests that higher losses of carbon will occur from soils in boreal and tundra regions in response to global warming.This is because these soils have the largest store of labile organic matter,and are predicted to experience the greatest rise in temperature7.Tropical soils may release less C than previously predicted4owing to a large store of SOM in deep soil8and the high proportion of resistant C pools in SOM.Soil warming experiments,an analogue for the effects of global warming on SOM decomposition9,suggest that the effect of warming on SOM decomposition may decline with time.The change in SOM composition associated with warming and the different temperature sensitivity of the C pools were assumed to be responsible for this decline10–11.Despite the common assertion that SOM composition affects the temperature sensitivity of SOM decomposition,experimental or modelling evidence is yet to be presented.If the temperature sensitivity of SOM decomposition is not affected by SOM composition,predictions of climate change impacts on soil stored C will be greatly affected.By definition,the temperature sensitivity of SOM decomposition is the change in SOM decomposition rate with temperature under otherwise constant conditions5.At present,this concept is often confused with concepts of SOM turnover4,12–13or SOM dynamics2–4 under different environmental conditions with accompanying different temperatures.Temperature sensitivity of SOM decompo-sition(or Q10)estimated by incubating soils at different but constant temperatures14–16or by radiocarbon accumulation in undisturbed soils13is confounded by many factors other than temperature.We incubated soil samples under changing temperature toletters to natureNATURE|VOL433|6JANUARY2005|/nature57©2005Nature Publishing Groupinvestigate the influence of SOM composition on the temperature dependence of SOM decomposition.Figure 1shows that soil C contents for both labile components (water-dissolved organic carbon (DOC),microbial carbon (C mic )and K 2SO 4-extracted carbon (C KSO ))and the total organic carbon (TOC),are signifi-cantly lower in the subsoil (20–30cm)than in the surface soil (0–10cm).The ratio of DOC:TOC and C KSO :TOC declined signifi-cantly with soil depth (F ¼28.5and 36.1,respectively,P ,0.0001),but C mic :TOC was not significantly affected by depth (F ¼1.9,P ,0.2).After the initial flush of CO 2emission,soil basal respi-ration rate at 208C was (mean ^s.e.m.)6.67^0.46m g CO 2per g dry soil per h for root-free samples in the 0–10cm layer,but only 1.92^0.20m g CO 2per g dry soil per h for the 20–30cm layer.Corresponding values were 6.27^0.66and 1.47^0.16for intact samples.Over a period up to 88days,the subsoil respired only ,0.29^0.13of the CO 2respired in the surface soil.These results indicate that soil basal respiration rate is closely related to variations in C pools occurring at different soil depths.Q 10values for individual soil samples varied in the range 1.97–2.21during the early stage of incubation (up to day 10).No significant correlation was found between Q 10and the rate of basal respiration.Relationships in Fig.1between respiration rate,Q 10value and SOM pools reflect the long-term acclimation of the microbial community to the environment (such as temperature,moisture and O 2)associated with soil depths.During the incubation,there was a significant decline in the labile components (Fig.2c,d,f).After 108days incubation,DOC was 0.73^0.14and C KSO was 0.62^0.065of initial values when averaged over all samples.The greatest variation following incu-bation was observed in C mic .The average C mic at day 42was only 0.43^0.13of the initial content,and less than 0.10^0.0057after 108days incubation.Changes in the average TOC during incu-bation were not significant (Fig.2b).At the end of the incubation,average TOC was 0.94^0.19of the initial content.Soil respiration rate consistently declined with time (Fig.2e).The association between respiration rate and C mic during the incubation suggests that the variation in microbial biomass may be a major cause of the temporal changes in soil respiration.The response of soil basal respiration to temperature was notaffected by the depletion of labile C during the incubation.Q 10values averaged for all samples were in the range 2.01–2.30for the whole incubation period (Fig.2a).There is no significant change in Q 10for soil basal respiration with incubation time,despite the fact that Q 10was more variable during the later stages of incubation.As time progressed,the resistant C component contributed a greater portion of the total soil basal respiration owing to the depletion of labile C pools (Supplementary Fig.2).The Q 10value for soil basal respiration should gradually decrease if resistant C is significantly different from labile pools and insensitive to temperature variation (Supplementary Fig.3).A constant Q 10for soil basal respiration suggests that the temperature dependence of resistant C is not significantly different from that for labile pools.In most incubation experiments,soil samples have been sepa-rately incubated at different but constant temperatures 12,14,15.Three different methods have been used to estimate SOM decomposition and its temperature sensitivity:the total mass loss 3,17,the time required for a given percentage of mass loss 17,and the soil respir-ation rate 14,18.A decline in soil respiration rate was commonly observed as incubation times increased 14,17,19,20.This decline is expected to be greater at higher than at lower temperatures because of the greater depletion and degradation of C pools 21.Temperature sensitivity is likely to be underestimated if turnover rate is derived from studies of total mass loss for a given time period or from respiration rates at different constant temperatures,owing to the higher decline in C turnover rate at higher temperature.If Q 10is estimated using the time required for a given percentage of mass loss,the value will be overestimated.In this case,temporal effects on estimated C turnover rate are more pronounced at lower temperatures than at higher temperatures.Data of total mass loss from soil incubations longer than one year were used to support the opinion that decomposition rates of organic matter in mineral soil do not vary with temperature 4.Estimated C turnover rates from a long-term incubation will be significantly different from those occurring in the field,owing to the quick decline in soil microbial biomass and respiration rate during incubation.In such exper-iments,the temperature sensitivity of SOM decomposition may have been seriously biased or underestimated because respiration rates at all temperatures are close to zero at the later stage of incubation.In soil warming experiments,the observed decline of warming effects on SOM decomposition with time 11does not necessarily mean that the decomposition of resistant C is less sensitive to elevated temperature than the labile component.Provided that the increase in net primary production (NPP)due to warming islessFigure 1Soil carbon components,respiration rate and associated Q 10values with respect to soil depth and sampling method (four replicates for each sample).Respiration rate was an average of data measured at 208C in days 3and 5.The Q 10value was estimated with soil respiration rates under changing temperature for the period of days 3–10.All values are normalized against that of surface root-free sample.Soil respiration rate is significantly related to concentrations of C pools owing to soil depth andsampling method,but Q 10does not change with respiration rate or C concentrations.Error bars indicate standard deviation.DOC,dissolved organic carbon;TOC,total organiccarbon.Figure 2Variations in respiration rate and soil carbon pools with increasing incubation time.Values are averages of all four samples,and normalized by initial values.a ,Q 10value;b ,TOC;c ,DOC;d ,K 2SO 4-extracted C;e ,respiration rate at 208C;and f ,microbial biomass C.Error bars are standard deviation.Respiration rate declined rapidly owing to the depletion of labile components (DOC,C KSO and C mic ),but the Q 10value of soil respiration remained unchanged.letters to natureNATURE |VOL 433|6JANUARY 2005|/nature58© 2005Nature Publishing Groupthan the increase in SOM decomposition rate,a decline in warming effect on SOM decomposition is always expected.In the long-term, the microbial community may become acclimated to warming with changed activities.The contribution of this acclimation is not yet clear.For long-term climate change,the response of the resistant pool of SOM plays a critical role in regulating soil C stocks.Given the predicted climate change in Europe in the next century,the greatest loss of SOM is expected in soils where the present mean annual temperature(MAT)is less than48C,and this net release of SOM will gradually decrease with MAT gradient(Fig.3a–c).(This predicted climate change is climate forcing according to the implementation by the Hadley Centre Climate Model(HadCM3) of the Intergovernmental Panel on Climate Change(IPCC)A1FI (world market–fossil fuel intensive)emission scenario22.)With a moderate change in the temperature sensitivity of the resistant C pool(humus pool of the Rothamsted Carbon Model23only),from Q10¼2.98to about2.58(at108C),sensitivity induced change will significantly reduce the net SOM release in temperate soils(present MAT.48C).By2100,the reduction in SOM loss could be up to 46%in arable soil,37%in grassland,and32%in forest for regions where the present MAT is greater than158C(Fig.3d).At the global scale,this reduction will be large enough to change our prediction of the magnitude and spatial pattern of SOM stocks in the future.Our study does not support the opinion that resistant C pools are significantly less responsive to temperature variation than labile C pools.A MethodsSoil samples(intact and root-free)were collected from a middle-aged plantation of Sitka spruce(Picea sitchensis)in Scotland(568370N,38480W).Mineral soils were collected from four locations in the site at depths of0–10,20–30cm.Root-free samples were made by sieving soil through a2mm mesh to remove plant detritus,root and gravel.For each depth,approximately600–800g soil was taken and packed into a chamber to the original bulk density.Intact soil samples(,10£10cm)were taken next to each root-free sample, following the method of ref.5.Soil samples were analysed to determine TOC24,DOC25and C KSO26.C mic was determined by fumigation extraction26.Samples(16in total)were incubated in the laboratory using a programmable water bath(developed in The University of Edinburgh,UK).Temperature was changed commonly between4and448C (continuously increased from the lowest to the highest with a step of48C and then decreased,reaching a new temperature within two hours).Each temperature was held for about9h.Before and after each round of temperature change,soils were kept at208C for a few days.Soil moisture contents were monitored and adjusted accordingly by adding water at the surface of the soil sample,and fresh air was continuously passed through each chamber during the incubation.Respired CO2was measured with an infrared gas analyser in differential mode,logged every second for7min for each chamber,but only the average over the last four minutes was used.The16chambers were measured sequentially,and four rounds of measurement were made before changing to another temperature.During each round of temperature change,the mean respiration rate at a given temperature was an average of values measured at that temperature when the temperature was increasing and decreasing(Supplementary Fig.1).Mean respiration rates at different temperatures werefitted with an exponential model5ðR¼a exp½ln Q10ðT=10Þ Þto calculate the Q10 value.More information about data analysis is included in the Supplementary Methods, which also explain how we assessed contributions of the resistant C pool to the total SOM decomposition and its Q10.Received30July;accepted22October2004;doi:10.1038/nature03138.1.Lenton,T.M.&Huntingford,C.Global terrestrial carbon storage and uncertainties in its temperaturesensitivity examined with a simple model.Glob.Change Biol.9,1333–1352(2003).2.Liski,J.,Ilvesniemi,H.,Ma¨kela¨,A.&Westman,C.J.CO2emissions from soil in response to climaticwarming are overestimated—The decomposition of old soil organic matter is tolerant of temperature.Ambio28,171–174(1999).3.Thornley,J.H.M.&Cannell,M.G.R.Soil carbon storage response to temperature:a hypothesis.Ann.Bot.87,591–598(2001).4.Giardina,C.P.&Ryan,M.G.Evidence that decomposition rates of organic carbon in mineral soil donot vary with temperature.Nature404,858–861(2000).5.Fang,C.&Moncrieff,J.B.The dependence of soil CO2efflux on temperature.Soil Biol.Biochem.33,155–165(2001).6.Sanderman,J.,Amundson,R.G.&Baldocchi,D.D.Application of eddy covariance measurements tothe temperature dependence of soil organic matter mean residence time.Glob.Biogeochem.Cycles17, doi:10.1029/2001GB001833(2003).7.Schlesinger,W.H.&Andrews,J.A.Soil respiration and the global carbon cycle.Biogeochemistry48,7–20(2000).8.Jobba´gy,E.G.&Jackson,R.B.The vertical distribution of soil organic carbon and its relation toclimate and vegetation.Ecol.Appl.10,423–436(2000).9.Rustad,L.E.et al.A meta-analysis of the response of soil respiration,net nitrogen mineralization,andaboveground plant growth to experimental ecosystem warming.Oecologia126,543–562(2001). 10.Peterjohn,W.T.,Melillo,J.M.&Bowles,S.T.Soil warming and trace gasfluxes:experimental designand preliminaryflux results.Oecologia93,18–24(1993).11.Peterjohn,W.T.et al.Response of trace gasfluxes and N availability to experimentally elevated soiltemperature.Ecol.Appl.4,617–625(1994).12.Dalias,P.,Anderson,J.M.,Bottner,P.&Couˆteaux,M.-M.T emperature responses of carbonmineralization in conifer forest soils from different regional climates incubated under standard laboratory conditions.Glob.Change Biol.6,181–192(2001).13.Trumbore,S.E.,Chadwick,O.A.&Amundson,R.Rapid exchange between soil carbon andatmospheric carbon dioxide driven by temperature change.Science272,393–396(1996).14.Winkler,J.P.,Cherry,R.S.&Schlesinger,W.H.The Q10relationship of microbial respiration in atemperate forest soil.Soil Biol.Biochem.28,1067–1072(1996).15.MacDonald,N.W.,Zak,D.R.&Pregitzer,K.S.Temperature effects on kinetics of microbialrespiration and net nitrogen and sulfur mineralization.Soil Sci.Soc.Am.J.59,233–240(1995). 16.Ross,D.J.&Tate,K.R.Microbial C and N,and respiratory activity,in litter and soil of a southernbeech(Nothofagus)forest:distribution and properties.Soil Biol.Biochem.25,477–483(1994). 17.Reichstein,M.,Bednorz,F.,Broll,G.&Ka¨tterer,T.T emperature dependence of carbon mineralisation:conclusions from a long-term incubation of subalpine soil samples.Soil Biol.Biochem.32,947–958 (2000).18.Fierer,N.,Allen,A.S.,Schimel,J.P.&Holden,P.A.Controls on microbial CO2production:acomparison of surface and subsurface soil horizon.Glob.Change Biol.9,1322–1332(2003).19.Lovell,R.D.&Jarvis,S.C.Soil microbial biomass and activity in soil from different grasslandmanagement treatments stored under controlled conditions.Soil Biol.Biochem.30,2077–2085 (1998).20.Lomander,A.,Ka¨tterer,T.&Andre´n,O.Carbon dioxide evolution from top-and subsoil as affected bymoisture and constant andfluctuating temperature.Soil Biol.Biochem.30,2017–2022(1998). 21.Grisi,B.,Grace,C.,Brookes,P.C.,Benedetti,A.&Dell’abate,M.T.Temperature effects on organicmatter and microbial biomass dynamics in temperate and tropical soils.Soil Biol.Biochem.30, 1309–1315(1998).22.IPCC.Special Report on Emissions Scenarios(Cambridge Univ.Press,Cambridge,UK,2000).23.Coleman,K.&Jenkinson,D.S.in Evaluation of Soil Organic Matter Models Using Existing Long-TermDatasets(eds Powlson,D.S.,Smith,P.&Smith,J.U.)237–246(NATO ASI Series I Vol.38,Springer, Heidelberg,1996).24.Allen,S.E.,Grimshaw,H.M.,Parkingson,J.A.&Quarmby,C.Chemical Analysis of EcologicalMaterials137–139(Blackwell Scientific,Oxford,1974).25.Martin-Olmedo,P.&Rees,R.M.Short-term N availability in response to dissolved organic-carbonfrom poultry manure,alone or in combination with cellulose.Biol.Fert.Soils29,386–393(1999).26.O¨hlinger,R.in Methods in Soil Biology(eds Schinner,F.et al.)56–58(Springer,Berlin,1995). Supplementary Information accompanies the paper on /nature. Acknowledgements We thank M.Wattenbarch and C.Zhang for assistance with the modelling. The pan-European modelling used data sets arising from the EU-funded ATEAM project. Competing interests statement The authors declare that they have no competingfinancial interests.Correspondence and requests for materials should be addressed to C.F.(c.fang@).Figure3Changes in soil C by2100for European soils.The baseline(solid lines in a–c)was from the original Roth-C model23projection(Q10¼2.98at108C for all C pools).Thetemperature sensitivity of humus was changed to80%of the original value(Q10¼2.58at108C,dashed lines in a–c).The loss of soil C is an average of all grid cells(21,976cellsat100£100resolution)according to present MAT.The percentage of net soil C loss withmodified Q10¼2.58for humus is relative to baseline decreases with MAT gradient(d).SOM,soil organic matter.letters to natureNATURE|VOL433|6JANUARY2005|/nature59©2005Nature Publishing Group。
csle流失方程
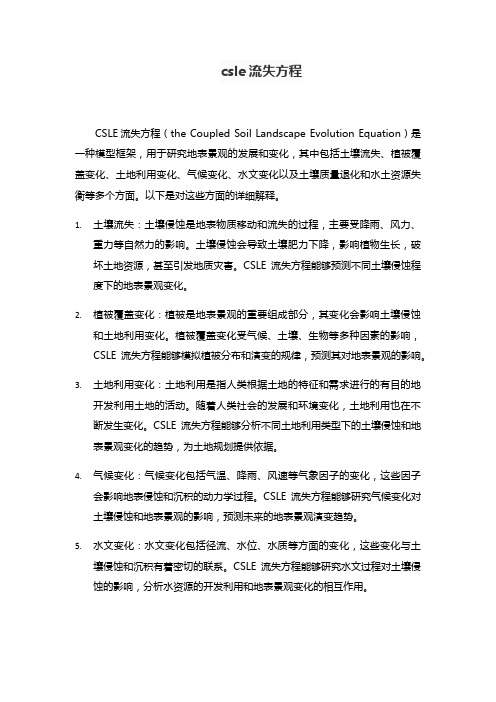
csle流失方程CSLE流失方程(the Coupled Soil Landscape Evolution Equation)是一种模型框架,用于研究地表景观的发展和变化,其中包括土壤流失、植被覆盖变化、土地利用变化、气候变化、水文变化以及土壤质量退化和水土资源失衡等多个方面。
以下是对这些方面的详细解释。
1.土壤流失:土壤侵蚀是地表物质移动和流失的过程,主要受降雨、风力、重力等自然力的影响。
土壤侵蚀会导致土壤肥力下降,影响植物生长,破坏土地资源,甚至引发地质灾害。
CSLE流失方程能够预测不同土壤侵蚀程度下的地表景观变化。
2.植被覆盖变化:植被是地表景观的重要组成部分,其变化会影响土壤侵蚀和土地利用变化。
植被覆盖变化受气候、土壤、生物等多种因素的影响,CSLE流失方程能够模拟植被分布和演变的规律,预测其对地表景观的影响。
3.土地利用变化:土地利用是指人类根据土地的特征和需求进行的有目的地开发利用土地的活动。
随着人类社会的发展和环境变化,土地利用也在不断发生变化。
CSLE流失方程能够分析不同土地利用类型下的土壤侵蚀和地表景观变化的趋势,为土地规划提供依据。
4.气候变化:气候变化包括气温、降雨、风速等气象因子的变化,这些因子会影响地表侵蚀和沉积的动力学过程。
CSLE流失方程能够研究气候变化对土壤侵蚀和地表景观的影响,预测未来的地表景观演变趋势。
5.水文变化:水文变化包括径流、水位、水质等方面的变化,这些变化与土壤侵蚀和沉积有着密切的联系。
CSLE流失方程能够研究水文过程对土壤侵蚀的影响,分析水资源的开发利用和地表景观变化的相互作用。
6.土壤质量退化:土壤质量退化是指土壤肥力下降、土壤结构破坏、土壤污染等方面的现象。
这些现象会影响植物生长和土地生产力,进而影响人类生存和发展。
CSLE流失方程能够评估不同土地利用方式和环境因素下的土壤质量退化程度,为土壤保护提供科学依据。
7.水土资源失衡:水土资源失衡是指水资源和土地资源的数量和质量不能满足人类需求的现象。
施氮对高寒垂穗披碱草草地根际、非根际土壤肥力的影响
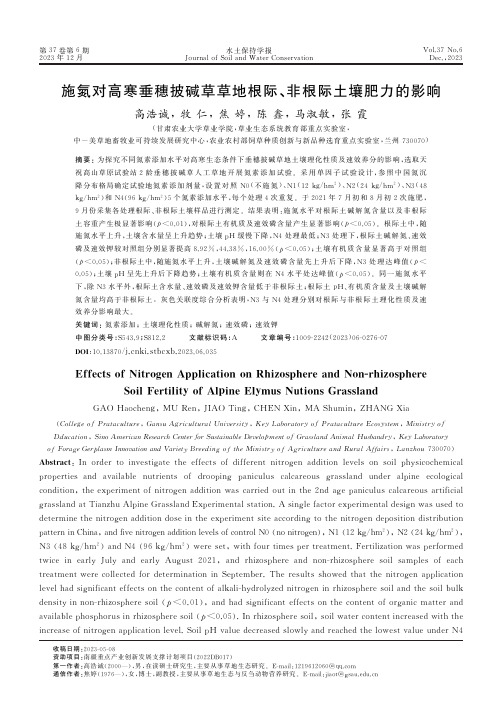
第37卷第6期2023年12月水土保持学报J o u r n a l o f S o i l a n d W a t e rC o n s e r v a t i o nV o l .37N o .6D e c .,2023收稿日期:2023-05-08资助项目:南疆重点产业创新发展支撑计划项目(2022D B 017) 第一作者:高浩诚(2000 ),男,在读硕士研究生,主要从事草地生态研究㊂E -m a i l :1219612060@q q .c o m 通信作者:焦婷(1976 ),女,博士,副教授,主要从事草地生态与反刍动物营养研究㊂E -m a i l :j i a o t @g s a u .e d u .c n施氮对高寒垂穗披碱草草地根际㊁非根际土壤肥力的影响高浩诚,牧仁,焦婷,陈鑫,马淑敏,张霞(甘肃农业大学草业学院,草业生态系统教育部重点实验室,中-美草地畜牧业可持续发展研究中心,农业农村部饲草种质创新与新品种选育重点实验室,兰州730070)摘要:为探究不同氮素添加水平对高寒生态条件下垂穗披碱草地土壤理化性质及速效养分的影响,选取天祝高山草原试验站2龄垂穗披碱草人工草地开展氮素添加试验㊂采用单因子试验设计,参照中国氮沉降分布格局确定试验地氮素添加剂量,设置对照N 0(不施氮)㊁N 1(12k g /h m 2)㊁N 2(24k g/h m 2)㊁N 3(48k g /h m 2)和N 4(96k g/h m 2)5个氮素添加水平,每个处理4次重复㊂于2021年7月初和8月初2次施肥,9月份采集各处理根际㊁非根际土壤样品进行测定㊂结果表明:施氮水平对根际土碱解氮含量以及非根际土容重产生极显著影响(p <0.01),对根际土有机质及速效磷含量产生显著影响(p <0.05)㊂根际土中,随施氮水平上升,土壤含水量呈上升趋势;土壤p H 缓慢下降,N 4处理最低;N 3处理下,根际土碱解氮㊁速效磷及速效钾较对照组分别显著提高8.92%,44.38%,16.00%(p <0.05);土壤有机质含量显著高于对照组(p <0.05);非根际土中,随施氮水平上升,土壤碱解氮及速效磷含量先上升后下降,N 3处理达峰值(p <0.05);土壤p H 呈先上升后下降趋势;土壤有机质含量则在N 4水平处达峰值(p <0.05)㊂同一施氮水平下,除N 3水平外,根际土含水量㊁速效磷及速效钾含量低于非根际土;根际土p H ㊁有机质含量及土壤碱解氮含量均高于非根际土㊂灰色关联度综合分析表明,N 3与N 4处理分别对根际与非根际土理化性质及速效养分影响最大㊂关键词:氮素添加;土壤理化性质;碱解氮;速效磷;速效钾中图分类号:S 543.9;S 812.2 文献标识码:A 文章编号:1009-2242(2023)06-0276-07D O I :10.13870/j.c n k i .s t b c x b .2023.06.035E f f e c t s o fN i t r o g e nA p p l i c a t i o no nR h i z o s p h e r e a n dN o n -r h i z o s ph e r e S o i l F e r t i l i t y o fA l p i n eE l ym u sN u t i o n sG r a s s l a n d G A O H a o c h e n g ,MU R e n ,J I A O T i n g,C H E N X i n ,MAS h u m i n ,Z H A N G X i a (C o l l e g e o f P r a t a c u l t u r e ,G a n s uA g r i c u l t u r a lU n i v e r s i t y ,K e y L a b o r a t o r y o f P r a t a c u l t u r eE c o s y s t e m ,M i n i s t r y o fD d u c a t i o n ,S i n oA m e r i c a nR e s e a r c hC e n t e r f o rS u s t a i n a b l eD e v e l o p m e n t o f G r a s s l a n dA n i m a lH u s b a n d r y ,K e y L a b o r a t o r yo f F o r a g eG e r p l a s mI n n o v a t i o na n dV a r i e t y B r e e d i n g o f t h eM i n i s t r y o f A g r i c u l t u r e a n dR u r a lA f fa i r s ,L a n z h o u 730070)Ab s t r ac t :I no rde rt oi n v e s t i g a t et h eef f e c t so fd i f f e r e n tn i t r og e na d d i t i o nl e v e l so ns o i l ph ys i c o c h e m i c a l p r o p e r t i e sa n d a v a i l a b l e n u t r i e n t s o f d r o o p i n g p a n i c u l u s c a l c a r e o u s g r a s s l a n d u n d e r a l p i n e e c o l o gi c a l c o n d i t i o n ,t h e e x p e r i m e n t o fn i t r o g e na d d i t i o nw a s c a r r i e do u t i nt h e2n da g e p a n i c u l u s c a l c a r e o u sa r t i f i c i a l g r a s s l a n da tT i a n z h uA l p i n eG r a s s l a n dE x p e r i m e n t a l s t a t i o n .As i n g l e f a c t o r e x p e r i m e n t a l d e s i g nw a s u s e d t o d e t e r m i n e t h en i t r o g e na d d i t i o nd o s e i n t h e e x p e r i m e n t s i t e a c c o r d i n g t o t h en i t r o g e nd e p o s i t i o nd i s t r i b u t i o n p a t t e r n i nC h i n a ,a n d f i v e n i t r o g e n a d d i t i o n l e v e l s o f c o n t r o l N 0(n o n i t r o g e n ),N 1(12k g /h m 2),N 2(24k g /h m 2),N 3(48k g /h m 2)a n dN 4(96k g/h m 2)w e r e s e t ,w i t h f o u r t i m e s p e r t r e a t m e n t .F e r t i l i z a t i o nw a s p e r f o r m e d t w i c ei n e a r l y J u l y a n d e a r l y A u g u s t2021,a n dr h i z o s p h e r ea n d n o n -r h i z o s p h e r es o i ls a m p l e so fe a c h t r e a t m e n tw e r e c o l l e c t e df o rd e t e r m i n a t i o n i nS e p t e m b e r .T h er e s u l t ss h o w e dt h a t t h en i t r o g e na p p l i c a t i o n l e v e l h a d s i g n i f i c a n t e f f e c t s o n t h e c o n t e n t o f a l k a l i -h y d r o l y z e dn i t r o g e n i nr h i z o s ph e r e s o i l a n d t h e s o i l b u l k d e n s i t y i nn o n -r h i z o s p h e r e s o i l (p <0.01),a n dh a ds i g n i f i c a n t e f f e c t so nt h e c o n t e n t o f o r g a n i cm a t t e r a n d a v a i l a b l e p h o s p h o r u s i n r h i z o s p h e r e s o i l (p <0.05).I n r h i z o s p h e r e s o i l ,s o i lw a t e r c o n t e n t i n c r e a s e dw i t h t h e i n c r e a s e o f n i t r o g e na p p l i c a t i o n l e v e l .S o i l p Hv a l u e d e c r e a s e d s l o w l y an d r e a c h e d t h e l o w e s t v a l u eu n d e rN 4t r e a t m e n t.U n d e r N3t r e a t m e n t,a l k a l i-h y d r o l y t i cn i t r o g e n,a v a i l a b l e p h o s p h o r u sa n da v a i l a b l e p o t a s s i u m i n r h i z o s p h e r e s o i lw e r e s i g n i f i c a n t l y i n c r e a s e db y8.92%,44.38%a n d16.00%c o m p a r e dw i t h c o n t r o l g r o u p (p<0.05).S o i l o r g a n i cm a t t e r c o n t e n tw a s a l s o s i g n i f i c a n t l y h i g h e r t h a n t h a t i n c o n t r o l g r o u p(p<0.05).I n n o n-r h i z o s p h e r e s o i l,w i t h t h e i n c r e a s eo f n i t r o g e na p p l i c a t i o n l e v e l,s o i l a l k a l i-h y d r o l y t i cn i t r o g e na n da v a i l a b l e p h o s p h o r u s c o n t e n t s i n c r e a s e d f i r s t a n d t h e nd e c r e a s e d,a n d t h e p e a kv a l u ew a s r e a c h e du n d e rN3t r e a t m e n t (p<0.05).S o i l p Hv a l u e i n c r e a s e d f i r s t a n d t h e nd e c r e a s e d.T h e c o n t e n t o f s o i l o r g a n i cm a t t e r r e a c h e d t h e p e a kv a l u e a t t h e l e v e l o fN4(p<0.05).U n d e r t h es a m en i t r o g e na p p l i c a t i o n l e v e l,t h ec o n t e n t so fw a t e r c o n t e n t,a v a i l a b l e p h o s p h o r u s a n d a v a i l a b l e p o t a s s i u m i n r h i z o s p h e r e s o i l w e r e l o w e r t h a n t h o s e i n n o n-r h i z o s p h e r e s o i l e x c e p tN3l e v e l.p H,o r g a n i cm a t t e r c o n t e n t a n da l k a l i-h y d r o l y z e dn i t r o g e nc o n t e n t o f r h i z o s p h e r e s o i lw e r e h i g h e r t h a n t h o s e o f n o n-r h i z o s p h e r e s o i l.T h e c o m p r e h e n s i v e a n a l y s i s o f g r e y c o r r e l a t i o nd e g r e e s h o w e d t h a t N3t r e a t m e n ta n d N4t r e a t m e n t s h a dt h e g r e a t e s te f f e c t s o n p h y s i c o c h e m i c a l p r o p e r t i e sa n d a v a i l a b l e n u t r i e n t s o f r h i z o s p h e r e a n dn o n-r h i z o s p h e r e s o i l,r e s p e c t i v e l y.K e y w o r d s:n i t r o g e na d d i t i o n;p h y s i c a l a n dc h e m i c a l p r o p e r t i e so f s o i l;a l k a l i h y d r o l y z e dn i t r o g e n;a v a i l a b l e p h o s p h o r u s;a v a i l a b l e p o t a s s i u m我国草地资源非常丰富,天然草地约400ˑ108 h m2,承担着涵养水源以及保护生物多样性等重要作用,维护着生态系统的平衡[1],且在我国农业㊁畜牧业㊁旅游业㊁制造业及餐饮业等多方面创造着巨大的经济价值㊂随着人们对草地资源的过度利用,我国草地快速退化,约90%的天然草地处在不同程度的退化进程之中,随之而来的是草地资源快速减少㊂由于高寒草地生态系统对于外界干扰极其敏感,同时自身阻抗及恢复能力较差,全国的高寒草地生境状况逐步恶化,祁连山区尤为明显㊂祁连山高寒草地超载率达69.1%,退化率46.9%㊂根据李海云[2]对祁连山高寒草地退化中 植物 土壤 微生物 的研究表明,土壤理化性质直接影响草地生态系统的演替过程,同时也在草地生态系统退化过程中受到不同程度的影响㊂土壤中氮素㊁磷素等营养元素的含量是衡量土壤是否退化以及退化程度的重要指标,土壤中的速效养分含量也随着高寒草地的退化程度不同而发生不同的变化㊂对于正在退化或退化程度较高的土壤进行施氮处理可有效减缓其退化速度㊂胡冬雪[3]研究表明,土壤中的有机质与碱解氮含量均随草地施氮而增加,土壤p H㊁土壤容重及土壤含水量等指标无显著变化;武建双等[4]对高寒草地人工垂穗披碱草种群对施氮处理的响应的研究表明,高寒草地土壤p H均不随施氮处理变化而发生变化㊂土壤氮素含量随施氮量增加而增加㊂施用氮肥可为土壤有效供给氮素,并且能提髙土壤中速效氮含量;李盼盼[5]研究表明,土壤有机质含量随施氮量增加而增加,呈现正相关关系,而速效磷含量却与施氮量呈负相关,土壤全氮含量无显著变化,但大量添加氮素也不利于改善草地退化问题;宗宁等[6]研究表明,高水平的氮肥与磷肥配施反而造成轻度退化草地群落物种的丰富度和均匀度的显著降低,加剧土壤退化程度㊂因此,探索一个合适的施氮水平,使其既能改善土壤退化问题,又保证草地生态系统稳定,是一个亟待解决的问题㊂根际是指受植物根系活动的影响后,与原土体在理化特性以及生物学特性上有显著差异的特殊土壤区域,是植物㊁土壤㊁微生物及环境因子进行相互作用的场所,是各类养分㊁水分㊁有益和有害物质及生物作用于根系或进入根系参与物质循环的重要区域,其自身具备特殊的微生态系统㊂根际微域环境对土壤养分能否被植物有效利用以及其被植物根系利用效率有直接影响,并与植物的抗逆能力强弱㊁根系病害的预防与抵抗以及农林业持续发展等方面密切相关[7]㊂根际土对植株生长的影响明显强于其他土体,根际土中的养分含量普遍大于非根际,根际土与土体其他部分所含养分含量也有显著差异㊂与非根际土相比,根际土养分含量以及微生物数量均高于前者,各种物质循环和能量转化较非根际土而言更为迅速,土壤微生物活性更强[8]㊂同时,曹永昌等[9]研究表明,植物根际附近多数大多数酶的酶活性多要高于非根际土中酶活性㊂通过对根际土㊁非根际土理化性质㊁土壤养分含量及土壤酶活性等的对比,可建立一个更直观与准确的土壤质量评价体系㊂因此,根际㊁非根际土在不同施氮水平下,土壤速效养分含量变化的状况可能存在非常显著的差异㊂垂穗披碱草(E l y m u s n u t a n s G r i s e b)是禾本科多年生丛生草本植物,因其植株生长茂盛㊁粗蛋白含量高的特性被广泛用于高寒退化草场的改良以及人工草地的建设,是修复高寒退化草地和建植人工草地的首选草种㊂本试验通过不同水平的外源氮素人772第6期高浩诚等:施氮对高寒垂穗披碱草草地根际㊁非根际土壤肥力的影响工添加处理,探讨在不同氮素添加水平下高寒草地根际㊁非根际土理化性质及速效养分的变化,以期为高寒草地种植垂穗披碱草地合理施加氮肥提供理论依据㊂1材料与方法1.1研究区概况研究区位于甘肃省武威市天祝藏族自治县甘肃农业大学天祝高山草原生态系统试验站2龄垂穗披碱草人工草地(36ʎ30' 37ʎ35'N,102ʎ17' 103ʎ40'E)㊂海拔2960m,气候寒冷,年均气温0ħ,年均降水量400mm,年蒸发量1398mm,生长期130天㊂试验地土壤为山地黑钙土,有机质㊁全氮㊁全磷㊁全钾含量分别为12%,0.77%,0.11%,2.14%,水解氮㊁速效钾含量分别为496,330m g/g㊂1.2试验设计采用单因子设计,通过参照中国氮沉降分布格局[10],确定甘肃地区干湿沉降率为8.16k g/(h m2㊃a),从而确定试验氮素添加量,即将氮添加设置对照N0 (不施氮)㊁N1(12k g/h m2)㊁N2(24k g/h m2)㊁N3(48 k g/h m2)和N4(96k g/h m2),等同于甘肃地区自然氮沉降量1,5,3,6,12倍㊂即尿素施加量分别为0,52.17, 104.35,208.70,417.40g㊂以N0作为对照,2020年5月开始种植垂穗披碱草,播种量为22.5k g/h m2㊂将试验地(29mˑ19m)划分为20个面积为4mˑ5m 的小区,为防止不同试验处理间互相干扰,在每个小区之间设立宽度为1m的保护行㊂试验采取完全随机区组设计,设5个施肥水平,每个水平4次重复㊂经计算不同施氮水平所需的氮添加后,称取出每个试验组所需尿素,将其充分溶解于2L的水中㊂在无风的天气下,将不同处理所需尿素溶液利用喷壶在各试验小区之间进行均匀喷洒,同时在C K小区喷洒施等量的水,以便统一草地植物生长环境标准㊂在2021年7月初和8月初进行施肥,分别为全年施肥量的50%,此期间不定期清除杂草且不浇水㊂1.3取样方法在垂穗披碱草建植后,分别采集根际土和非根际土㊂每个试验小区随机选取3个采样点,每个采样点以20c m样段为基准把垂穗披碱草植株及根系挖出,去掉浮土,采用抖落法收集根际土,装入保鲜袋,用冰袋低温保存根际土样本,带回实验室进行测定㊂每个试验小区中随机选取5个点,每个点分别采集0 10,10 20,20 30c m土层土样,将每层5个点的土样混合均匀,去除土层中根系及杂质,用四分法取150g左右称重,采集的土壤立即进行保鲜处理,带回实验室,保存方法同根际土㊂施肥第2年,2021年蜡熟期(8月中旬)对土壤根际土与非根际土采用以上相同方法进行采样㊁保鲜处理㊂带回实验室测定后与垂穗披碱草建植初期的根际土㊁非根际土速效养分进行对比分析㊂1.4指标测定参考鲍士旦[11]对土壤理化性质及速效养分含量进行测定,土壤p H采用玻璃电极法进行测定;土壤含水量采用恒温烘干法测定;土壤容重采用环刀法测定;土壤有机质含量采用重铬酸钾外加热法测定;土壤速效氮含量采用N a O H碱解扩散法测定;土壤速效磷含量采用碳酸氢钠浸提钼蓝比色法测定;土壤速效钾含量采用醋酸铵-火焰光度计法测定㊂1.5数据统计及分析采用E x c e l2016软件进行数据处理和图表制作,用S P S S27.0软件进行单因素(o n e-w a y A N O-V A)㊁最小显著差异法(L S D法)方差分析和多重比较(α=0.05),图表中数据用平均值ʃ标准误表示㊂采用双因素方差分析(t w o-w a y A N O V A)施氮水平对垂穗披碱草草地根际与非根际土速效养分的影响㊂用灰色关联度法对各处理进行综合分析,根据关联度分析原则,关联度值越大,综合评价越好㊂假设参考数列为x0,比较数列为x i,i=1,2,3, ,n,且x0={x0(1),x0(2),x0(3), ,x0(n)},x i={x i(1), x i(2),x i(3), ,x i(n)},则称ζi(k)为x0与x i在第k点的关联系数,计算公式为:ξk=m i n i m i n k x0(k)-x i(k)+ρm a x i m a x k x0(k)-x i(k)x0(k)-x i(k)+ρm a x i m a x k x0(k)-x i(k)式中:x0(k)-x i(k)表示在k点x0数列和x i数列的绝对差值,记作Δi(k),其中m i n i m i n k x0(k)-x i(k)为二级最小差;m a x i m a x k x0(k)-x i(k)为二级最大差;ρ为分辨系数,取值范围为0~1,一般取ρ=0.5㊂将关联系数ξk代入公式:r i=1nðn k=1ξ(k),算出关联度值㊂2结果与分析2.1施氮水平对根际、非根际土理化性质的影响由表1方差分析可知,施氮水平对根际土碱解氮含量产生极显著影响(p<0.01);对土壤有机质及速效磷含量产生显著影响(p<0.05);施氮水平对非根际土容重产生极显著影响(p<0.01)㊂施氮水平对于根际土与非根际土中含水量㊁p H及速效钾含量以及非根际土有机质㊁碱解氮㊁速效磷含量均未产生显著影响㊂872水土保持学报第37卷表1 施氮水平对根际土㊁非根际土理化性质与速效养分影响的方差分析因素土壤类型含水量pH 有机质容重碱解氮速效磷速效钾施氮水平根际土1.6562.9414.728*8.592**4.674*1.891非根际土2.7451.9142.5837.440**3.0290.5910.691注:**㊁*分别表示p <0.01,p <0.05㊂下同㊂2.2 不同施氮水平对根际㊁非根际土中含水量及p H的影响由表2可知,随着施氮量增加,N 1处理下根际土含水量较未施氮处理显著降低10.00%(p <0.05);各处理间非根际土含水量无明显变化;根际土p H 缓慢下降,N 4处理下达最低值,非根际土p H 整体呈先上升后下降趋势㊂相同施氮水平下,非根际土含水量均大于根际含水量;土壤p H 表现为非根际土小于根际土㊂表2 不同施氮水平对根际土㊁非根际土含水量及p H 的影响施氮水平土壤含水量/%根际土非根际土土壤p H根际土非根际土N 00.20ʃ0a0.23ʃ0b 7.98ʃ0.02a 7.96ʃ0.02a b N 10.18ʃ0b0.23ʃ0a b 7.99ʃ0.02a 7.96ʃ0.02a b N 20.19ʃ0a b0.22ʃ0b 7.97ʃ0.02a 7.98ʃ0.02a N 30.19ʃ0.01a b 0.23ʃ0.01b7.96ʃ0.03a 7.91ʃ0.03bN 40.20ʃ0.01a b0.24ʃ0a7.80ʃ0.09b7.96ʃ0.01a b注:表中数据均为平均值ʃ标准差;同列不同小写字母表示同一土壤类型下不同施氮水平之间差异显著(p <0.05)㊂下同㊂2.3 不同施氮水平对根际㊁非根际土有机质及非根际土容重的影响由表3可知,随施氮水平增加,N 3处理下根际土有机质较未施氮处理提高15.18%(p <0.05),总体呈上升趋势;非根际土有机质含量则在N 4水平处达到最大,显著高于未施氮处理(p <0.05);N 4处理下土壤容重达到峰值(p <0.05)㊂在相同施氮水平下,土壤有机质表现为根际土大于非根际土㊂表3 不同施氮水平对根际土㊁非根际土有机质及非根际土容重的影响施氮水平土壤有机质/(m g ㊃k g -1)根际土非根际土土壤容重/(g ㊃c m -3)非根际土N 0117.59ʃ2.59b 104.72ʃ1.03b1.10ʃ0.01b N 1124.32ʃ1.36b 107.83ʃ1.30a b 1.08ʃ0.01b N 2126.60ʃ8.21b 108.06ʃ1.15a b 1.12ʃ0.00b N 3144.54ʃ3.60a 108.22ʃ0.81a b 1.09ʃ0.01b N 4126.10ʃ4.14b109.57ʃ1.21a1.15ʃ0.01a2.4 不同施氮水平对根际㊁非根际土速效养分影响2.4.1 不同施氮水平对根际㊁非根际土碱解氮的影响 由图1可知,随施氮量增加,N 3处理下根际土和非根际土碱解氮含量均最高,显著大于未施氮处理(p <0.05);除对照组外,同一施氮水平下,根际土碱解氮含量均有大于非根际土趋势㊂注:图柱上方不同小写字母代表在同一施氮水平下不同土层之间差异显著(p <0.05)㊂下同㊂图1 不同施氮水平对根际土㊁非根际土碱解氮含量的影响2.4.2 不同施氮水平对草地根际㊁非根际土中速效磷的影响 由图2可知,随施氮水平增加,N 3处理下根际土㊁非根际土速效磷含量同时达最大值,分别较未施氮处理提高44.38%和8.43%(p <0.05)㊂除N 3处理外,同一施氮水平下,土壤速效磷表现为根际土小于非根际土㊂图2 不同施氮水平对草地根际㊁非根际土速效磷的影响2.4.3 不同施氮水平对草地根际㊁非根际土速效钾的影响 由图3可知,随着施氮水平提升,非根际土速效钾含量在各处理间差异不显著;N 3处理下根际土速效钾含量显著高于其他处理(p <0.05),且除N 3水平外,土壤速效钾含量均表现为根际土小于非根际土㊂图3 不同施氮水平对草地根际㊁非根际土速效钾的影响972第6期 高浩诚等:施氮对高寒垂穗披碱草草地根际㊁非根际土壤肥力的影响2.5不同施氮水平对于根际㊁非根际土理化性质与速效养分灰色关联度综合分析为进一步探究不同施氮水平对垂穗披碱草地根际土㊁非根际土的影响,采用灰色关联度综合分析法,对不同施氮水平下根际㊁非根际土理化特性以及速效养分进行综合分析㊂由表4可知,根际土关联度排序为N3> N1>N2>N4>N0,即N3处理对于垂穗披碱草地根际土理化性质及速效养分影响效果最佳;非根际土关联度排序为N4>N3>N2>N0>N1,即N4处理对于垂穗披碱草地非根际土理化性质及速效养分影响效果最佳㊂表4不同施氮水平对于根际㊁非根际土理化性质与速效养分灰色关联度综合分析土壤类型因素含水量p H有机质容重碱解氮速效磷速效钾关联度排名N01.000.990.450.650.330.520.665N10.651.000.520.940.490.630.702根际土N20.720.990.550.840.420.660.703 N30.840.971.001.001.001.000.971N40.850.870.550.930.400.530.694N00.450.960.530.490.530.390.830.604N10.530.950.760.050.660.630.520.585非根际土N20.401.000.780.600.630.471.000.703 N30.430.840.800.481.001.000.460.722N41.000.961.001.000.650.330.670.8013讨论3.1不同施氮水平对根际㊁非根际土理化性质影响权国玲等[12]研究表明,施肥对土壤容重㊁总孔度及含水量无显著影响,原因可能是土壤结构及理化性质相对稳定,仅进行短期施肥处理无法改变土壤理化性质㊂本研究中,随着施氮水平的上升,根际土含水量呈先下降后上升趋势,非根际土含水量呈略微上升趋势㊂试验结果与权国玲等[12]研究有略微不符,推断其原因可能为试验中氮肥添加是以尿素水溶液形式进行喷施,垂穗披碱草在吸收氮素时对土壤中水分起到一定的聚集作用,所以N1处理下根际土水分被连带吸收进入植物体内,而高氮处理下,垂穗披碱草根际需要吸收的尿素溶液随浓度升高而减少,因此,根际土含水量呈现逐渐回升现象㊂而非根际土受到垂穗披碱草根系对土壤水分吸收的作用较小,因此,含水量无明显变化㊂而在相同施氮水平下,非根际土含水量均低于根际土含水量,此现象也与垂穗披碱草根系对土壤中水分的主动吸收有关,根系在吸收水分时,在一定程度上将水分聚集到根系附近的土壤中,造成根际土含水量高于非根际土含水量㊂土壤容重的大小同时受到土壤质地㊁结构㊁有机质含量以及各种因素的共同影响,能够粗略地反映土壤质地以及持水透气状况,是衡量及判断土壤结构的重要指标之一㊂本试验中,非根际土容重无明显变化,但在N4水平下达到最大㊂张雪丽[13]研究认为,外源氮素添加引起土壤发生化学侵蚀,导致土壤结构发生改变,进而使土壤容重受到影响,出现容重增加或降低的现象㊂同时外源氮素的不断输入造成土壤腐殖质持续分解和流失,导致土壤紧实度提高,造成土壤容重增加的现象[14]㊂本试验中,N4水平下土壤容重显著增加,其余施氮处理均无明显变化㊂由于N1㊁N2及N3施氮水平在短期不足以改变土壤容重,而在N4水平下,外源氮添加已经引起土壤结构改变,同时使得土壤腐殖质开始分解流失,与土壤有机质在N3水平下达到最高,而N4水平处开始下降的现象相符㊂所以非根际土容重在N4水平下达到最大,与前文权国玲等[12]㊁张雪丽[13]研究相符㊂土壤有机质含量是评价土壤肥力的核心指标之一,土壤有机质含量越高,对土壤结构的促成作用越明显,对土壤自身所具备的吸收能力提升越强,对土体的缓冲性造成更积极影响,能为植物提供更多所需的营养成分㊂武运涛等[15]研究认为,土壤有机质根据其密度或粒径大小被分为颗粒态有机质(P OM)和矿质结合态有机质(MA OM),随着氮添加量的增加, P OM的氮含量随施氮量增加而显著增加;同时黄晶等[16]研究表明,适度施加氮肥能提高土壤有机质含量㊂本试验中,随施氮水平上升,根际土有机质在N3处理下达到最大,非根际土有机质含量也随施氮水平提升而逐渐上升,在N4水平处达到最大㊂相同施氮水平下,土壤有机质均表现为根际土大于非根际土㊂其原因推测为垂穗披碱草根系对于土壤中养分的吸收以及根系分泌物导致根际土中微生物含量高于非根际土㊂同时土壤微生物可通过利用植物残体的方式对土壤碳库进行补充[17],而土壤中的垂穗披碱草082水土保持学报第37卷根系残体或是土壤中本身具有的植物残体经过各种微生物分解后提升土壤有机质含量[18]㊂土壤p H可直观反映土壤酸碱性,对于衡量土壤是否适合作物栽培起到重要参考意义,并且土壤p H及容重与土壤有机质呈现显著的负相关关系[19]㊂即使土壤对于p H的改变具有一定的缓冲能力,但是长期外源氮素添加仍导致土壤p H下降[20]㊂根据刘贺永等[21]对模拟氮沉降对草地土壤p H和电导率的影响研究表明,土壤p H具有随氮添加量增加而下降的趋势,而土壤电导率与氮素添加水平呈现负相关关系㊂本研究中,随施氮水平上升,根际土p H缓慢下降,并且在N4水平处达到最低㊂而周细红等[22]研究表明,尿素对土壤p H影响大致可分为2个阶段,第1阶段中施用尿素可使土壤p H 增高,其原因在于尿素在水解过程中消耗土壤中的氢㊂但出现最大值后,随即进入第2阶段,土壤p H 开始随施氮水平上升而降低㊂其原因为土壤中尿素水解产生的N H+4可以通过土壤微生物转化为亚硝酸或硝酸盐,并产生H+,导致土壤p H下降㊂本研究中,非根际土p H在N2水平处达到最大随后下降,整体呈先上升后下降趋势,此结果与周细红等[22]研究结论相似㊂同时,土壤有机质含量对提升土壤缓冲性能,减少土壤p H波动具有重要作用㊂而本试验中土壤p H表现为非根际土小于根际土,仅在N4水平处比根际土p H高出2.01%㊂原因推测为非根际土的尿素未被植物吸收已被水解转化,产生H+,降低土壤p H,而根际土中的尿素被根系吸收一部分,所以产生的H+少,根际土p H下降相对较缓㊂3.2不同施氮水平对根际、非根际土速效养分的影响碱解氮由无机态氮和结构简单且能被直接吸收利用的有机态氮组成,可供作物直接吸收快速利用,故又称为速效氮㊂通过对草地进行外源氮添加可显著增加土壤速效氮含量㊂本试验中,随着施氮水平提升,根际土碱解氮含量逐渐上升,在N3水平下达到最大;非根际土碱解氮含量先上升后下降,在N3水平下达到最大,总体呈上升趋势,与晏和飘[23]研究结果一致㊂在同一施氮水平下,根际土碱解氮含量在未施氮处理下低于非根际土含量,在N1㊁N2㊁N3及N4下根际碱解氮含量均高于非根际土碱解氮含量㊂此现象原因为在未施氮处理下,植物根系对附近土壤中碱解氮的吸收㊂在外源氮素添加之后,植物根系对于碱解氮的主动吸收使得部分尿素聚集在根系附近,导致土壤中碱解氮含量提升,高于非根际土碱解氮含量㊂速效磷是当季作物从土壤中主要吸收的磷元素来源,在一定程度上可反映土壤对作物的磷素供应水平㊂夏昊[24]在黔西南州烤烟氮素调控技术研究发现,速效磷含量随着施氮水平提升先增高后降低;同时宋春燕等[25]研究表明,由于氮素对磷的吸收有一定的协同作用,作物氮素的吸收促进对速效磷和速效钾的利用,所以速效磷含量随氮施用量增多而下降㊂本试验中,随着施氮水平提升,根际土速效磷含量逐渐上升,在N3水平下达到最大后下降,总体呈略微上升趋势㊂N3水平下根际土速效磷含量显著高于未施氮处理㊁N2及N4水平㊂非根际土速效磷含量呈先上升后下降趋势,在N3水平下达到最大,与以上研究结果一致㊂在同一施氮水平下,除N3水平外,根际土速效磷含量均低于非根际土含量㊂此现象是因为垂穗披碱草根系对于速效磷的吸收导致根系附近土壤中速效磷含量低于距离根系稍远的非根际土速效磷含量,与前文结论相符㊂而在N3水平下,根际土速效磷含量高于非根际土含量,与前文结论不符,推测原因为试验条件与试验地基况不一致,导致试验结果有差异㊂施加N3水平尿素的试验地速效磷含量可能较为充足,经施加氮肥后,根际土速效磷含量与非根际土速效磷含量得以同时提升㊂随着施氮水平提升,根际土速效钾含量略微上升,在N3水平下达到最大后迅速下降,总体无明显变化;非根际土中速效钾含量无明显变化;在同一施氮水平下,根际土速效钾含量低于非根际土速效钾含量㊂此现象原因为垂穗披碱草根系对于土壤中氮素的吸收促进对速效磷和速效钾的利用,导致根系附近的速效钾含量始终低于远离根系的非根际土,与宋春燕等[25]研究结论一致㊂结合不同氮素添加水平对高寒生态条件下垂穗披碱草人工草地土壤理化性质及速效养分影响进行综合分析讨论㊂同时,对于不同施氮水平下根际㊁非根际不同速效养分的不同响应状况,应从土壤综合性质及土壤速效养分之间的相互作用来综合分析㊂试验采用灰色关联度分析法对氮素添加水平㊁土壤理化性质及速效养分含量进行综合分析发现,48k g/h m2处理对于垂穗披碱草地根际土理化性质影响效果最佳,96k g/h m2处理对于垂穗披碱草地非根际土理化性质影响效果最佳㊂4结论(1)施氮可改善垂穗披碱草草地土壤理化性质和提高土壤速效养分含量㊂(2)施氮水平对根际土碱解氮含量以及非根际土容重产生极显著影响,对根际土有机质㊁速效磷含量产生显著影响㊂182第6期高浩诚等:施氮对高寒垂穗披碱草草地根际㊁非根际土壤肥力的影响。
农田生态系统微生物多样性研究方法及应用

收稿日期:2008-05-13基金项目:中国科学院创新项目(KZCX2-YW-407);中国科学院野外台站基金项目和国家科技支撑计划课题(2006BAD05B01作者简介:毕明丽(1984-),女,在读硕士,从事土壤肥力与养分循环研究。
E-mail:bi-yours@ *通讯作者:E-mail:wtyu@农田生态系统微生物多样性研究方法及应用毕明丽,宇万太*(中国科学院沈阳应用生态研究所,辽宁沈阳110016)摘要:微生物在农田生态系统中占据着很重要的作用,其结构和功能的多样性及变化在一定程度上反映了农田生态系统的基本状态,因此非常有必要应用有效的方法来研究农田微生物的多样性、分布及行为等。
但是通过传统的微生物培养和鉴定方法得到的微生物信息很片面,不足以代表微生物在农田生态系统中的真实情况。
而近几十年来兴起的微生物研究新方法突破了传统方法的限制,极大地促进了微生物学的发展。
本文介绍了现在常用的微生物多样性研究方法及其在农田生态系统中的应用现状。
关键词:微生物多样性;研究方法;分子生物学;农田生态系统中图分类号:S153.6文献标识码:A文章编号:0564-3945(2009)06-1460-07Vol.40,No.6Dec.,2009土壤通报Chinese Journal of Soil Science第40卷第6期2009年12月微生物是农田生态系统的重要组成部分,在动植物残体分解、养分循环、氮的固定、土壤结构与肥力保持和病虫害防治等方面都起着很重要的作用[1],微生物的多样性和群落结构在一定程度上反映了农田生态系统的基本状况,保持微生物的生态过程和多样性是农业生产赖以生存的基础。
通过研究农田生态系统中微生物的分布和多样性,探讨农田生态系统的影响因素及其作用机理,可以为今后制定农业管理计划提供科学依据,具有很重要的理论和实践意义。
农田生态系统中微生物种类极其丰富,文献记载1g 农田土壤中就含有几百万细菌、数十万真菌孢子、数万个原生动物和藻类[2],它们在农田生态系统中起着至关重要的作用。
plant and soil

REGULAR ARTICLEEffects of NO3−-N on the growth and salinity tolerance of Tamarix laxa WilldXiaodong Ding&Changyan Tian&Shirong Zhang&Jie Song&Fusuo Zhang&Guohua Mi&Gu FengReceived:20October2009/Accepted:8November2009/Published online:5December2009#Springer Science+Business Media B.V.2009Abstract The influence of NO3−-N on growth and osmotic adjustment was studied in Tamarix laxa Willd.,a halophyte with salt glands on its twigs. Seedlings of xa Willd.were exposed to1mM (control)or300mM NaCl,with0.05,1or 10mM NO3−-N for24days.The relative growth rate of seedlings at300mM NaCl was lower than that of control plants at all NO3−-N levels,but the concentrations of organic N and total N in the twigs did not differ between the two NaCl treatments. Increasing NO3−supply under300mM NaCl im-proved the growth of xa,indicating that NO3−played positive roles in improving salt resistance of the plant.The twigs of xa Willd.accumulated mainly inorganic ions,especially Na+and Cl−,to lower osmotic potential(Ψs):the contributions of Na+ and Cl−toΨs were estimated at31%and27% respectively,at the highest levels of supply of both NaCl and NO3−-N.The estimated contribution of NO3−-N toΨs was as high as20%in the twigs in these conditions,indicating that NO3−was also involved in osmotic adjustment in the twigs.Further-more,increases in tissue NO3−were accompanied by decreases in tissue Cl−and proline under 300mM NaCl.The estimated contribution of proline toΨs declined as with NO3−-N supply increased from 1to10mM,while the contributions of nitrate toΨs were enhanced under300mM NaCl.This suggested that higher accumulation of nitrate in the vacuole alleviated the effects of salinity stress on the plant by balancing the osmotic potential.In conclusion,NO3−-N played both nutritional and osmotic roles in xa Willd.in saline conditions.Keywords Tamarix laxa willd.Salt stress.NO3−-N. Osmoregulation.ProlineIntroductionSalinity reduces plant growth through osmotic stress, ion toxicity,and consequently nutritional stress (Nublat et al.2001).Many studies showed that improving of the nutritional status of plants by nitrogen or phosphorus fertilizers may mitigate the negative impacts of increased salinity and promote thePlant Soil(2010)331:57–67DOI10.1007/s11104-009-0231-7Responsible Editor:John McPherson Cheeseman.X.Ding:S.Zhang:F.Zhang:G.Mi:G.Feng(*) College of Resource and Environmental Science, China Agricultural University;Key Laboratory of Plant Nutrition,MOA;Key Laboratory of Plant-Soil Interactions,MOE, Beijing100193,People’s Republic of Chinae-mail:fenggu@C.TianXinjiang Institute Ecology and Geography,Chinese Academy of Sciences,Urumqi830011,People’s Republic of ChinaJ.SongCollege of Life Science,Shandong Normal University, Jinan250014,People’s Republic of Chinagrowth of plants(Ebert et al.2002;Papadopoulos and Rendig1983).Such a role is believed as an indirect effect of nitrogen on salinity resistance in plants.Nitrogen(N)shortage is one of the main factors limiting plant growth in many ecosystems,particular-ly in saline soils(Albassam2001;Botella et al.1997). Some saline soils,however, e.g.nitric saline soil (belonging to the Typic Salorthids)in Turpan Basin, Xinjiang,northwestern China,contain nitrate concen-tration as high as2–16g kg−1in0–30cm soil layer (Wang et al.1993;Yang1999).Several plant species grow in these nitric saline soils,such as Tamarix spp., Phragmites australis L.,Suaeda pterantha(Kar.et Kir.)Beg,Kalidium foliatum(Pall.)Moq,Halopeplis pygmaea(Pall.)Bge,Saliconia europaea L., Halostachys caspica(Bieb.)C.A.Mey.,Artemisia anethifolia Web.ex Stechm,Aeluropus pungens(M. Bieb.)C.Koch.,and Karelinia caspica(Pall.)Less (Xi et al.2006).Previous research on the relationship between N and salt resistance in plants has mainly focused on the assimilation of N and/or the secondary metabolism of N-containing compounds.For example,increased N supply can promote salt resistance in plants by accumulation of soluble N containing organic com-pounds(e.g.,proline,glycinebetaine and free amino acids)under salinity stress(Dubey and Pessarakli 1995).The possible role of these compatible solutes is in protecting the plants against photoinhibition (Hayashi et al.1997;Holmström et al.2000;Yang et al.2007),reactive oxygen species(Demiral and Türkan2004;Heidari and Mesri2008)or osmotic stress in plants growing under salt stress(Hasegawa et al.2000;Shen et al.1999;Yancey2005).Salinity decreases NO3−uptake by roots(Martinez and Cerda1989),inhibits the activity of nitrate reductase(Campbell1988),and reduces NO3−trans-location from roots to shoots(Gouia et al.1994).On the other hand,salinity stress tends to result in an increase accumulation of proline(Moghaieb et al. 2004;Parida and Das2005)in non-halophytic plants (Albassam2001)and also in halophytes(Khan et al. 2000),and the levels of nitrogenous solutes rises as N supply increases,e.g.,Spartina alterniflora(Colmer et al.1996).NO3−is the main form of N uptake by angiosperms (Martinoia et al.1981).When NO3−absorption exceeds reduction capacity,the excess NO3−will be stored in vacuoles(Blom-Zandstra and Lampe1983).Some ecological factors,e.g.light intensities(Blom-Zandstra and Lampe1985)or osmotic stress(Ourry et al.1992),may suppress the activity of nitrate reductase and result in NO3−accumulation in vacuoles.Marschner(1986)had hypothesized that the storage of NO3−in vacuoles might play a role in osmotic regulation when plants were growing in osmotic stress.However,previous studies have mainly focused on the nutritional function of NO3−, just a few studies have been conducted to assess Marschner’s hypothesis by testing the direct contri-bution of NO3−to the salt resistance of plants,and the results varied.For example,Stienstra(1986)showed that NO3−did not have a specific function in osmotic adjustment in Aster tripolium L.,a halophytic plant, grown in a nutrient solution with either a continuous or an intermittent NO3−supply.By contrast,Song et al.(2006a)suggested that NO3−not only has a nutritional role for growth of the euhalophyte Suaeda physophora,but also directly contributes to osmotic adjustment.In order to test Marschner’s hypothesis,a recretohalophyte(a halophyte with salt excretion glands),Tamarix laxa,was evaluated in hydroponic culture under controlled conditions,with three NO3−treatments under low(1mM)and high(300mM) NaCl-salinity.Materials and methodsPlant materialBranches of xa Willd.of0.5±0.1cm in diameter were collected in November2006,from Fukang, Xinjiang,China(44°18′N,87°55′E).Plant samples were chopped into10–12cm segments,dipped in water for24h,sterilized with0.5%potassium permanganate for30min,and then washed with tap water.The basal part of each segment was dipped in 0.1%rooting powder(ABT Rooting Powder®,pro-duced by ABT Research and Development Center of Chinese Academy of Forestry,Beijing,China)for 10h.The segments were then cultured in quartz sand for rooting and supplied initially with tap water. When new twigs appeared,the plants were cultured with1/10strength nutrient solution for30d and then with1/2strength nutrient solution renewed every 2days for another40days.The nutrient solution composition at full strength was:0.05mM Ca(NO3)2,2.95mM CaCl2,1mM K2SO4,2mM MgSO4, 1mM KH2PO4,90μM Fe-EDTA,46μM H3BO3, 9.1μM MnCl2,0.32μM CuSO4,0.76μM ZnSO4, 0.56μM Na2MoO4,1mM NaCl.The pH was adjusted each day to6.5±0.1with KOH or H2SO4. The pots were in a greenhouse with a light intensity of 480μmol m−2s−1,and temperature of30±3°C in day and24±3°C in night.The relative humidity was50–60%.In order to prevent salt accumulation in the quartz sand,relatively large volumes of fresh nutrient solution was used to irrigate the pots to leach out any excess salts every2days.Experimental designPlants were washed with deionized water,then transferred into1/2strength nutrient solution for 7days.The fresh weight of each seedling was measured,and similar-sized seedlings with fresh weight5.0±0.2g were selected before the pretreat-ment.The experiment was arranged in a completely randomized design with two factors:(1)two salinity levels,1mM(control)and300mM NaCl(salinity);(2)three nitrogen levels,0.05,1and10mM NO3−-N supplied as Ca(NO3)2.The extra Ca2+concentration in different treatments was balanced by CaCl2.In order to avoid osmotic shock,300mM NaCl was applied gradually by adding50mM NaCl per day. The plants were cultured in2L porcelain pots.Each pot contained two seedlings.Three seedlings of similar fresh weight per treatment were collected for dry weight(DW)when the final salinity concentra-tions were reached(as the initial dry weight for calculating relative growth rate).There were six replicates for each treatment,in which three replicates were used for evaluating biomass,and the other three were used for determining physiological parameters. The experiment was terminated24days after final salinity concentrations were reached.The twigs and roots were separated,and fresh weight(FW)was recorded.A sub-sample of fresh twigs samples of each plant was frozen in liquid N2.The remaining plant tissue samples were dried in an oven at80°C for 72h and dry weights(DW)were measured.Water content(WC)was calculated as:(FW-DW)/DW.The concentrations of Na+,K+and organic N were measured in oven-dried samples.Osmotic potential, and the concentrations of Cl−,NO3−and proline,were measured in samples frozen in liquid N2.Determination of the relative growth rates of plantsPlants were sampled at the beginning of treatments and at the end of the experiment.Relative growth rate(RGR) was calculated using the equation(Botella et al.1997):RGR¼log e W2Àlog e W1ðÞ=t2Àt1ðÞNote:W1and W2represent plant fresh weight at harvest1(1day after the final salinity concentrations were reached)and harvest2(at the end of the experiment)respectively,over the harvest interval t1 to t2(1to24days).Determination of inorganic ions,proline,amino acids, and organic N in plantsFrozen plant material(vegetative branches and roots of xa)was extracted with boiling distilled water, and Cl−and NO3−were determined after the solution was filtered.NO3−was determined by the colorimetric method(Cataldo et al.1975)(UV-120-02Spectro-photometer,Shimadzu,Kyoto,Japan),and Cl−was determined by0.03mM AgNO3titration method, with5%K2CrO4as indicator.Frozen plant tissue was also ground in10%acetic acid,and the ninhydrin colorimetric method was used for the determination of amino acids(Moore and Stein1954),or the concentra-tion of proline(Troll and Lindsley1955).For cation determinations,about15-mg dry sample was put in a muffle stove to be ashed.The ash was dissolved in 0.1ml of concentrated nitric acid and then diluted to a volume of20ml with deionized water.The concen-trations of Na+and K+were determined by flame photometry(Model2655-00Digital Flame Analyzer, Cole-Parmer Instrument Company,Chicago,USA). Dry plant samples were ground,and analyzed for organic N using the Kjeldahl method(Shi1994).Determination of osmotic potential(Ψs)The frozen plant tissues were put into a syringe to thaw. The liquid squeezed from the plant tissues was analysed using a freezing point osmometer(Fiske210;Advanced Instruments Inc.,Norwood,MA,USA)to measure the ic value(the value reading from the instrument).The tissue osmotic potential of solutes was calculated as Ψs=–ic RT,where i is ionization constant of the solute, c is the molar concentration of the solute,R is theuniversal gas constant and T is the temperature in degrees Kelvin(Song et al.2006a).Vacuoles account for most of the volume of the twigs, particularly in recretohalophyte plants which have large water storage cells(Bosabalidis and Thomson1985; Thomson1975).For the present analyses,the volume of the cytoplasm is assumed to occupy10%of cells. Therefore we calculated the average concentration of each inorganic ion in vacuole or organic solute in cytoplasm by the content of each individual osmolyte and the tissue water content with the volume ratio of vacuole vs cytoplasm9:1.The osmotic potential(Ψ)of each individual osmolyte,such as Na+,K+,Cl−,NO3−, or proline,was calculated byΨ=-nRT/V,where n is the number of solute molecules,R is the universal gas constant,T is temperature in°K,and V is the volume in liter.Osmotic coefficients of the solutes in tissue water were assumed to equal1(Song et al.2006a).The estimated contributions of each individual solute(C es)to the tissue osmotic potential was calculated using the equation:C es(%)=Ψ/Ψs×100.Statistical analysisAll data were subjected to a two-way ANOVA using the SAS™software(SAS Institute Inc.1989). Treatment means were compared by least significant differences(LSD)at P=0.05.ResultsEffect of NO3−-N on the growth of xaunder salinity stressNo differences were observed in the growth of xa twigs growing at1and10mM NO3−-N at either NaCl level(P<0.05)(Table1).However,twig fresh weights were much lower when grown at 0.05mM NO3−-N under either NaCl level(Table1). There were no significant differences in root fresh weights among the three levels of NO3−-N under either NaCl levels(Table1).Root/twig ratios were significantly higher in plants grown at 0.05mM NO3−-N,compared to values for plants grown at1and10mM NO3−-N under either NaCl levels.These results indicated that increased NO3−supply improved twig growth to some degree regard-less of salinity level.The average twig growth of plants at300mM NaCl was reduced compared to the low salt treatment,at all NO3−-N levels(Table1).Twig growth at 0.05mM NO3was similar at both1and300NaCl but differed between the two salt levels at1and 10mM nitrate.Twig fresh weight under1mM NaCl at 1and10mM NO3−-N was121%and133%higher respectively compared to values obtained at 300mM NaCl,whereas average root fresh weightTable1Effects of NaCl and NO3−-N on the growth of root and twig,root/twig ratio and relative growth rate of xa seedlings. Plants were treated with1or300mM NaCl and0.05,1or10mM NO3−-N for24daysNaCl(mM)NO3−-N(mM)increments of f.wt(g/plant)Root/Twig RGR(g·g−1·day−1)Twig Root1mM0.05 3.90b a 1.39a0.36a0.083b111.64a 2.16a0.19b0.111a1013.07a 2.49a0.19b0.103aMean b9.54A 2.01A0.24B0.097A300mM0.05 3.08b 1.08a0.35a0.051b1 5.27a 1.60a0.30b0.094a10 5.60a 1.58a0.28b0.083aMean 4.65B 1.75A0.31A0.080BAll data are means of3replicationsIncrements of f.wt(g/plant)were calculated by fresh weight at the end of the experiment minus fresh weight at the beginning of treatments a Values marked with different letter represented significant difference at P=0.05level across all NO3−-N levels at a given NaCl level b Means value for each NaCl level.Means values marked with different capital letter indicate significant differences at P=0.05level between NaCl levelsunder 300mM NaCl did not differ from that at 1mM NaCl (Table 1).Compared with 1mM NaCl,the average root/twig ratio was increased under the 300mM NaCl treatment,but the average relative growth rate (RGR)decreased (Table 1).These results indicated that 1mM NO 3−-N was enough to support maximal twig growth of xa under high salinity.The concentrations of NO 3−-N,Cl −,Na +,K +in twigs and roots of xaNO 3−concentrations in twigs of xa were enhanced with increasing NO 3−-N supply under 1or 300mM NaCl (Fig.1a ).Similar trends of NO 3−concentrations were found in roots (Fig.1b ).Compared with plants growing at 1mM NaCl,300mM NaCl reduced the NO 3−concentration in twigs of plants growing at both 1mM NO 3−-N and 10mM NO 3−-N (P <0.05),but did not affect the NO 3−concentration in roots (Fig.1a,b ).The concentration of Cl −in the twigs decreased with increasing NO 3−-N under both NaCl level except at 10mM NO 3−-N and 300mM NaCl treatment where Cl −concentration showed no significant difference comparing to 1mM NO 3−-N and 300mM NaCl treatment (Fig.1c ).In roots,the concentrations of Cl −were significantly higher at 300mM NaCl than those in 1mM NaCl at all NO 3−-N levels (Fig.1d ).NaCl concentration (mM)NaCl concentration (mM)(m o l L -1 w a t e r i n t h e v a c u o l e )(m o l L -1 w a t e r i n t h e v a c u o l e )0.00.10.20.30.40.50.00.10.20.30.40.5abc10 mmol·L -1NO 3--N1 mmol·L -1NO 3--N 0.05 mmol·L -1NO 3--NFig.1NO 3−(a ,b )and Cl −(c ,d )concentrations in the vacuole in twigs and in roots of xa ,which were treated with 1or 300mM NaCl and 0.05,1or 10mM NO 3−-N for 24daysComparing to low NO 3−-N supply,medium and high NO 3−-N levels reduced Cl −concentration in root at 300mM NaCl,while no significant differences were observed between the three NO 3−-N levels at 1mM NaCl (Fig.1d ).These results implied that higher NO 3−-N supply reduced Cl −uptake.Salinity increased the concentration of Na +in both twigs and roots (Fig.2a,b ).At 1mM NaCl,the concentration of Na +in twigs and in roots did not differ with increasing NO 3−-N supply.At 300mM NaCl,the Na +concentration was higher at 0.05mM NO 3−-N than at 1or 10mM NO 3−-N (Fig.2a,b ).These results indicated that supply of higher NO 3−-N levels might have reduced theconcentrations of Na +in both twigs and roots under higher salinity.K +concentration both in twigs and in roots were not significantly different among NO 3−-N treatments under either NaCl level (Fig.2c,d ).Contents of total N,organic N,and NO 3−-N in twigs and roots of xa with increasing supply of NO 3−-N at 1and 300mM NaClThe contents of total N (N total ),organic N (N org )and NO 3−-N in both twigs and roots were all enhanced with increasing supply of NO 3−-N under both NaCl levels compared to the values at 0.05mM NO 3−-N(m o l L -1 w a t e r i n t h e v a c u o l e )0.00.10.20.30.40.51300NaCl concentration (mM)(m o l L -1 w a t e r i n t h e v a c u o l e )1300NaCl concentration (mM)ac10 mmol·L -1NO 3--N1 mmol·L -1NO 3--N0.05 mmol·L -1NO 3--NFig.2Effect of NO 3−-N supply on the concentrations of Na +(a ,b ),K +(c ,d )in the vacuole in twigs and roots of xa which were treated with 1or 300mM NaCl,with 0.05,1or 10mM NO 3−-N for 24dayssupply(Table2).In the high salinity treatment (300mN NaCl),the contents of either total N(N total), or organic N(N org)in twigs were similar between treatments of1and10mM NO3−-N,whereas NO3−-N contents in twigs were significantly different across all three NO3−-N levels at this salinityThe concentrations of total N(N total)and organic N in twigs were enhanced in the300mM NaCl treat-ment compared with those at1mM NaCl,whereas NO3−concentrations were reduced(P<0.05).Salinity had no significant effect on the concentrations of total N(N total)and NO3−-N in roots.Effect of NO3−-N supply on the osmotic potential (Ψs)and the estimated contribution(C es)of Na+, K+,Cl−and NO3−toΨs in twigs of xaThe NO3−-N supply had no effect on theΨs in twigs of xa,while salinity significantly reduced theΨs (Table3).The estimated contribution of Na+toΨs (C Na)decreased with increasing NO3−-N supply at 300mM NaCl(Table3).However,there were no significant differences in the C Na among the three NO3−-N levels at1mM NaCl.C Na increased with salinity,from about7.23%at1mM NaCl up to 35.78%at300mM NaCl(Table3).The estimated contribution of Cl−toΨs(C Cl) declined with the increasing NO3−-N at both NaCl level.The decrease in contribution of Cl−with increasing NO3−-N was more marked at1mM NaCl than at300mM although the interaction was not statistically significant(Table3).The estimated contribution of K+toΨs(C K) decreased with increasing NO3−-N supply at low salinity,but there were no significant differences among the different NO3−-N levels under300mM NaCl (Table3).C K decreased with increased NaCl supply.The estimated contribution of NO3−toΨs(C NO3−) increased with the increase of NO3−-N at both1mM and300mM NaCl,whereas it was lower at high salinity at any given NO3−level(Table3).Effect of NO3−-N supply on the concentrationand the estimated contribution of proline toΨsin twigs of xaBased on the assumption that proline was restricted to the cytosol,and that that accounted for10%of the total cell volume,there was a significant increase inTable2The effects of NO3−-N on the contents of total N,organic N and NO3−-N in twigs and roots of xa which were treated with1or300mM NaCl and0.05,1or10mM NO3−-N for24daysNaCl NO3−-N Ntotal(mg·g−1DW)Norg(mg·g−1DW)N NO3−(mg·g−1DW)(mM)(mM)Twig Root Twig Root Twig Root10.0520.88c d13.60b20.34b12.57b0.54c0.91b137.80b22.87a32.11a17.98a 5.69b 4.37a1043.50a25.94a34.72a20.59a8.79a 3.74aMean e34.06B f20.80A29.06B17.05B 5.01A 3.01A 3000.0526.51b16.26b25.60b15.38c 1.03c0.87b 139.80a25.25a35.42a19.82a 4.9b 5.44a1037.83a22.75a34.09a17.48b 5.34a 5.27aMean34.71A21.42A31.70A17.56A 3.76B 3.86A Analysis of Variance(F Values)Salinity(S)8.84b 1.27NS 6.62a 1.47NS10.96b0.77NS NO3−-N level(N)15.85c78.81c67.05c25.02c15.85c95.09cS×N 3.69NS8.61NS 2.94NS8.59NS8.71b 4.03NSa denotes significant difference at P=0.05,b denotes significant difference at P=0.01,c denotes significant difference at P=0.001,NS denotes no significant difference.Data represent F valuesd Within each column,values with different letter are significantly different at P=0.05level across all NO3−-N levelse Mean value for each NaCl levelf Mean values with different capital letter are significantly different at P=0.05level between NaCl levelsproline concentration at 300mM NaCl compared to the low salinity treatment.Concentrations of proline rose with increasing NO 3−-N supply at 1mM NaCl;However,at 300mM NaCl,concentrations of proline were maximum at 1mM NO 3−-N supply,which indicated that 1mM NO 3−-N supply was enough for structural growth;however,as the level of NO 3−-N supply increased to 10mM the concentration of proline decreased as more NO 3−were being stored in the vacuoles.The concentration of proline was the lowest at 0.05mM NO 3−-N which implied that the proline synthesis was limited because of nitrogen deficiency (Fig.3a ).The estimated contribution of amino acids to Ψs (C pro )increased with increasing NO 3−-N at 1mM NaCl.At 300mM NaCl,it increased significantly when the NO 3−-N supply was increased from 0.05to 1mM,but then dropped at 10mM NO 3−-N (Fig.3b ).In general,the estimated contribution of proline to Ψs (C pro )was considerably lower at both salinities and all three NO 3−-N levels compared with the inorganic solutes described above:the maximum contribution of proline accounted for only 3.6%of Ψs .DiscussionNitrogen is an essential nutrient for higher plants.Salinity may suppress the uptake and assimilation of nitrate in plants,which results in nutritional disorder and growth inhibition (Dluzniewska et al.2007;Marschner 1986).In the present study,high salinity reduced twig growth and the relative growth rate (RGR)of xa plants (Table 1).However,the average total nitrogen or organic nitrogen contents in plants grown under 300mM NaCl were higher than those grown under 1mM NaCl,while the average NO 3−concentration was lower at high NaCl (Table 2).Such results indicated that the uptake and assimilation of NO 3−in xa were not suppressed by high salinity.Increasing the nitrogen supply under either level of NaCl supply improved the RGR of xa (Table 1),which can be partly attributed to the nutritional role of N as many other researchers have concluded (Irshad et al.2008;Leidi et al.1992).In order to lower water potential,halophytes accumulate large amounts of inorganic ions in the vacuole and synthesize a relatively small amount of low molecular weight organic compounds to balanceTable 3The effects of NO 3−-N onthe osmotic potential (Ψs ),water content (WC),the estimated contribution of Na +(CNa +),K +(CK +),Cl −(CCl −)and NO 3−(C NO 3−)to osmotic potential in twigs of xa which were treated with 1or 300mM NaCl and 0.05,1or 10mMNO 3−-N for 24days NaCl NO 3−-N Ψs WCCNa +CCl −CK +C NO 3−(mM)(mM)(MPa)(ml·g −1DW)(%)(%)(%)(%)10.05−1.30a d 3.03c 7.52a 54.2a 20.72a 2.4b 1−1.25a 3.81b 7.85a 28.94b 21.77a 29.2a 10−1.61a 4.14a 6.32a 11.89c 16.47b 29.47a Meane−1.39A f3.66A 7.23B 24.00B 19.46A 15.44A 3000.05−1.71a 3.87a 42.16a 46.61a 12.57a 2.29c 1−1.48a 3.96a 34.03b 27.4b 15.99a 10.72b 10−1.55a 3.90a 31.14b 27.08b 14.12a 19.93a Mean−1.58B 3.91A 35.78A 33.7A 14.22B 10.31B Analysis of Variance (F Values)Salinity (S)0.16NS 10.67a 83.77c 56.07c 41.03c 35.53b NO 3−-N level (N)0.68NS 17.2c 1.37NS 4.20a 6.08a 61.01c S ×N0.03NS 14.51b0.97NS0.7NS3.96NS10.04cadenotes significant difference at P =0.05,b denotes significant difference at P =0.01,c denotes significant difference at P =0.001,NS denotes not significant difference.Data represent F valuesd Within each column,values with different letter are significantly different at P =-0.05level across all NO 3−-N levels e Mean value for each NaCl levelfMean values with different capital letter are significantly different at P =0.05level between NaCl levels3−on the osmotic potential (Ψs ),water content (WC),the estimated contribution of Na +(CNa +),K +(CK +),Cl −(CCl −)and NO 3−(C NO 3−)to osmotic potential in twigs of xa which were treated with 1or 300mM NaCl and 0.05,1or 10mM NO 3−-N for 24daysthe osmotic pressure in the cytoplasm (Hasegawa et al.2000;Zhao et al.2003).Song et al.(2006b )demonstrated that euhalophyte Suaeda physophora is able to compartmentalize inorganic ions,especially Na +in the vacuole,and synthesize a relatively small amount of organic solutes to balance the osmotic pressure in the cytoplasm.In the present study,xa accumulated Na +and Cl −in twigs under high salinity (Figs.1,2),which would lower osmotic potential in the twigs (Table 3).However,it is difficult to directly test the real concentration of the solutes:it was as-sumed that inorganic solutes were mainly distributed in vacuole and that organic solutes entirely accumu-lated in cytoplasm.In the present study,we estimated the concentrations of inorganic or organic solutes by tissue water volume and the general ratio of vacuole to cytoplasm.The distribution was based on the assump-tion,justified by anatomical studies,that the vacuole accounted for 90%and the cytoplasm and organelles for 10%of the overall cell volume (Di Martino et al.2003).Therefore the relative estimated-contribution of the inorganic or organic solutes to osmotic potential can be quantified (Silveira et al.2009).Although Marschner (1986)had hypothesized that NO 3−stored in vacuoles might play a role in osmotic regulation when plants were growing in osmotic stress,whether or not NO 3−plays this role and directly contributes to salt resistance of plants is still poorly understood.As noted in the introduction,the answer may depend on the species being considered.Our present study showed that NO 3−-N supply significantly enhanced the contribution of NO 3−to osmotic potential,from 2%to 20%in twigs of xa under high NaCl (Table 3).Such result suggested that in addition to the nutritional role,NO 3−accumulation in the vacuoles could play an important role in balancing the osmotic potential in xa under high salinity with adequate NO 3−-N supply.The interaction between Cl −and NO 3−may strongly affect the contribution of both anions to osmotic regulation in plant.The use of more NO 3−but less Cl −ions for osmotic adjustment may prevent Cl −toxicity in Suaeda physophora (Song et al.2006a ).Cl −present in the expanded leaves of certain species is associated with chlorosis and death,and these injuries occur even when the Na +concentration is low in the leaves (Greenway and Munns 1983).However,in our present study,more NO 3−but less Cl −or Na +might be accumulated in the vacuole for osmotic adjustment at higher NO 3−supply,compared with 1mM NO 3−.As NO 3−supply increased,the decrease in the estimated contribution of Cl −to osmotic potential in xa was compensated by an increase in that of NO 3−(Table 3).This can be attributed to competition between NO 3−and Cl −for transport systems,which are proposed to play significant roles in uptake or the xylem loading of NO 3−and Cl −(Cerezo et al.1997;Köhler and Raschke 2000).Twigsc cbaa b5101520253013001300NaCl concentration (mM)NaCl concentration (mM)P r o l i n e c o n c e n t r a t i o n(m m o l L -1w a t e r i n t h e c y t o p l a s m )0.01.02.03.04.05.0P e r c e n t (%)ab10 mmol·L -1NO 3--N1 mmol·L -1NO 3--N0.05 mmol·L -1NO 3--NFig.3Proline concentration (a )and the estimated contribution of proline to Ψs (b )in the cytoplasm in twigs of xa which were treated with 1or 300mM NaCl and 0.05,1or 10mM NO 3−-N for 24days。
爱果利丰

Scotts公司将加速在农业方面的成长
北美客户
• Lawns & Plant Food • Lawn Service • Controls • Smith & Hawken
国际用户
-UK/IRL -Western EU -Central EU
全球
•Global Pro - Europe/NA - Emerging Markets - Pro Grass Seeds
N
冬天: 无加热温室 ,避免使用尿素,最好NO3• 水培 (惰性介质) ? 避免使用尿素和NH4+ NO3- 最好 选择含尿素态氮高有助于叶片快速见绿。
提示 … Scotts WSF 肥料基于需要什么 样的配比含有最理想的氮形态 组合。
•
叶面施肥 ?
施用的氮形态会影响 :
植株生长状态 土壤或者介质pH
施用的氮影响生长类型
氮的形态影响植物的生长方式 氮 形 态 高含量的 NH4 +Urea (>25%) 生长类型 • 疯 长 • 叶片变大 • 茎拉长 • 植物变硬 • 生长紧凑 用 于
N
• 观叶植物 • 开花植物早期 • 暖季 &夏季 • 避免用在惰性介质(水耕栽培) •开花植物后期 •低温季节 &冬季 •惰性介质 •水培
为何说Agroleaf/Universol 产品能提供最 优化的营养系统?
养分的最佳平衡值是至关重要的
多年的产品研究,以及Scotts公司区域销 售经理和最终用户之间的良好互动,提供 了我们最丰富的经验,设计出最佳的配比。
Agroleaf/Universol中各种养分配比处 于最佳值 不会产生拮抗或协同作用 每个生长阶段,都有合适的产品和合理 的氮磷钾配比
土壤学名词
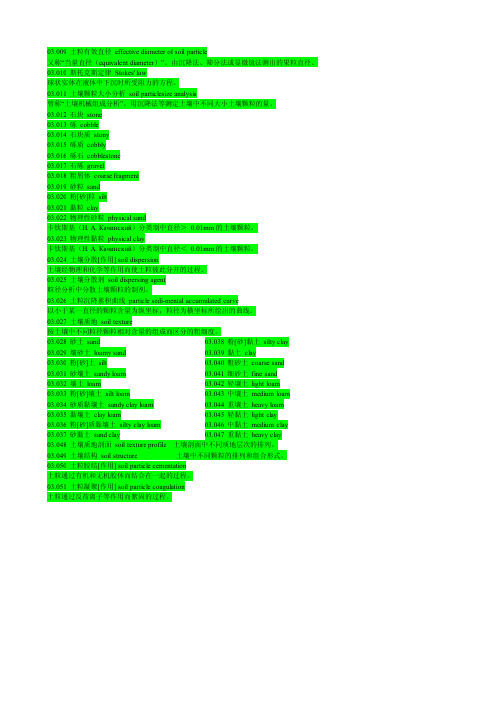
03.009土粒有效直径effective diameter of soil particle又称“当量直径(equivalent diameter)”。
由沉降法、筛分法或显微镜法测出的果粒直径。
03.010斯托克斯定律Stokes'law球状实体在液体中下沉时所受阻力的方程。
03.011土壤颗粒大小分析soil particlesize analysis曾称“土壤机械组成分析”。
用沉降法等测定土壤中不同大小土壤颗粒的量。
03.012石块stone03.013砾cobble03.014石块质stony03.015砾质cobbly03.016砾石cobblestone03.017石砾gravel03.018粗屑体coarse fragment03.019砂粒sand03.020粉[砂]粒silt03.021黏粒clay03.022物理性砂粒physical sand卡钦斯基(H.A.Качинский)分类制中直径>0.01mm的土壤颗粒。
03.023物理性黏粒physical clay卡钦斯基(H.A.Качинский)分类制中直径<0.01mm的土壤颗粒。
03.024土壤分散[作用]soil dispersion土壤经物理和化学等作用而使土粒彼此分开的过程。
03.025土壤分散剂soil dispersing agent粒径分析中分散土壤颗粒的制剂。
03.026土粒沉降累积曲线particle sedi-mental accumulated curve以小于某一直径的颗粒含量为纵坐标,粒径为横坐标所绘出的曲线。
03.027土壤质地soil texture按土壤中不同粒径颗粒相对含量的组成而区分的粗细度。
03.028砂土sand03.029壤砂土loamy sand03.030粉[砂]土silt03.031砂壤土sandy loam03.032壤土loam03.033粉[砂]壤土silt loam03.034砂质黏壤土sandy clay loam 03.035黏壤土clay loam03.036粉[砂]质黏壤土silty clay loam 03.037砂黏土sand clay 03.038粉[砂]黏土silty clay 03.039黏土clay03.040粗砂土coarse sand 03.041细砂土fine sand 03.042轻壤土light loam 03.043中壤土medium loam 03.044重壤土heavy loam 03.045轻黏土light clay 03.046中黏土medium clay 03.047重黏土heavy clay03.048土壤质地剖面soil texture profile土壤剖面中不同质地层次的排列。