Predictions of ground deformations in shallow tunnels in clay
上海软土地区某逆作法地铁深基坑变形

第51卷第8期2017年8月浙江大学学报(工学版)J o u rn a l o f Z h e jia n g U n iv e r s ity(E n g in e e rin g Science)V ol. 51 No. 8Aug. 2017D G1:10. 3785/j. issn. 1008-973X. 2017. 08. 007上海软土地区某逆作法地铁深基坑变形康志军12,黄润秋3,卫彬谭勇15(.同济大学地下建筑与工程系,上海200092;2.保利(成都)实业有限公司,四川成都610000;3.成都理工大学地质灾害防治与地质环境保护国家重点实验室,四川成都610059;.中铁二院华东勘察设计有限责任公司,上海200023;5.同济大学岩土及地下工程教育部重点实验室,上海200092)摘要:以上海软土地区某逆作法地铁车站深基坑项目为工程背景,通过分析现场监测数据,研究逆作法深基坑的变形性状及对周围环境的影响.研究结果发现:该基坑变形表现出显著的空间效应:中间标准段围护结构最大侧移的统计范围为(0.25%〜0. 45%)H,明显大于端头井的(0. 10%〜0.25%)H,中间标准段立柱隆起的上限为0.26%H,明显大于端头井的上限0. 18%H,中间标准段开挖引起的管线沉降明显大于端头井开挖引起的管线沉降;既有地下结构对基坑变形有明显的遮拦效应,导致中间标准段西侧的围护结构侧向变形较小;基坑开挖导致邻近浅基础建筑物发生较大的沉降,甚至破坏建筑物的结构整体性,引发墙体开裂;受软土流变特性的影响,浅基础建筑物和地下管线都产生一定程度的工后沉降.关键词:软土地区;逆作法深基坑;变形性状;空间效应;遮拦效应;土体流变中图分类号:T U447 文献标志码:A文章编号:1008 973X(2017)081527 10Deformation behaviors of deep top-down metro excavationin Shanghai soft clayK A N G Zhi-ju n1'2,H U A N G Run-qiu3,W E I Bin4,TAN Yon g''5(1. Department o f Geotechnical E n g in e e rin g,T o ng ji U niversity,Shanghai200092, C hina; 2. P oly (C H E N G D U)H o ldings Com pany L im ite d,C a e n p d u610000 »C hina t3. N atio nal Professional Laboratory o f GeologicaPrevention and Geological Environment Protection,Chengdu University o f Technology,Chengdu610059» C hina;4. China R a ilw a y Eryuan Engineering Group C o m p an y,East C hina Survey and Design lim ited C o m p a n y,S hanghai 200023,C hina; 5. Key Laboratory o f Geotechnical and U nderground Engineering o f M inistry o fE d ucation,T o ng ji U niversity,S hanghai200092, China)A b stra ct:The measured deform ation behaviors of the excavation and its influences on environm ent wereanalyzed based on fie ld instrum entation data from a top-dow n excavation in Shanghai sott clay.Resultsshowed that excavation behaviors exhibited apparent spatial corner effect.The m axim um lateral w alldeflections at the central standard segm entsw ere (0. 25 %〜0.45 %)H,greater than (0. 10 %〜0. 25 %)Hat end shafts.The upper bound of colum n u p lifts was around 0. 26 %H at the central standard segm ents,greater than0. 18%H at end shafts.The settlem ents of u tility pipelines near the central standard segmentswere greater to o.The existing underground structures adjacent to the west p it side imposed apparentbarrier effect on excavation d efo rm ations,i.e.,relatively sm aller lateral w a ll deflections we along the west p it side.Excavating induced significant settlem ents of adjacent buildings on shallow-收稿日期:2016 - 01 - 28. 浙江大学学报(工学版)网址:w w w. z;j--)u m a ls com/eng基金项目:国家重点研发计划资助项目(2016Y F C0800204)国家“973”重点基础研究发展计划资助项目(2015C B057800)国家自然科学 基金资助项目(1130745).作者简介:康志军(1991 —),男,硕士,主要从事深基坑工程等研究.G R C ID: 0000-0001-5540-2494. E-m a ld em rem g eo@通信联系人:谭勇,男,教授.G R C ID: 0000-0003-3J_07-5454. E-m ail: tan y ong2J_th@tongji. 1528浙江大学学报(工学版)第51卷foundation. The monitored wall cracking indicated that structural integrity of these buildings was damagedto different extents. Noticeable post-excavation settlements were observed at adjacent buildings and utilitypipelines, due to creeping of soft clay.Key words:soft clay; top-down excavation;deformation behavior;spatial corner effect; barrier ef creeping软土地区深基坑开挖引起土体应力状态改变,不可避免造成周围地层的移动,对周围环境产生不同程度的影响.在过去的几十年里,许多学者通过现场监测等 手段建立了一系列的经验法和半经验法评估软土基 坑开挖引起的围护结构变形、地表沉降、建筑物变形[-4].近年来,诸多学者利用各种研究手段对基坑开挖变形进行了各方面的研究.俞建霖等[5]用空间有 限单元法研究了基坑开挖过程中围护结构变形、周 围地表沉降、基坑底部隆起的空间分布;刘国彬等[6]对基坑工程进行了全方位的介绍;W a n g等[7]基于大量的现场监测数据研究了上海地区采用不同施工方案以及不同围护结构基坑的变形性状;T a n等[]发现:软土地区的地铁深基坑开挖至坑底后,及时浇筑混凝土底板能够有效抑制围护结构侧向变形和地表沉降的发展;T a n等9系统研究了上 海软土地层中顺作法基坑的变形性状及基坑几何形状与平面尺寸大小对开挖变形的影响;X u等[1<)]研究了周边超载对基坑变形的影响;郑刚等[11]通过 数值模拟研究了不同围护结构变形形式对周围建筑物变形的影响机理;徐长节等[12]利用有限元软件,分析了非对称开挖条件下基坑的变形性状;应 宏伟等[13]研究了坑外地下水位波动对软土地区基坑水土压力的影响机理.城市建筑密集区域的地铁车站深基坑工程需重点关注开挖对周边环境的影响.逆作法基坑采用 现浇混凝土楼板作为围护结构的水平支撑,能够增 大基坑支护系统的整体刚度,有效控制基坑变形、减少基坑开挖对周围环境的不利影响,逆作法工艺 被应用于城市中心地区的深基坑工程[1-15].本文依 托上海某逆作法地铁车站基坑工程,结合实际施工 过程,对现场监测数据进行分析,研究了软土地层中逆作法地铁车站深基坑的变形特点及对周边建筑物和地下管线的影响.1基坑周边环境本文的研究背景为位于上海商业区的某地铁车 站基坑项目,基坑平面布置如图1所示.基坑由南端 头井、中间标准段、北端头井3部分组成,基坑平面 尺寸为152 m X25 m,最大开挖深度为24〜26 m.基坑周边有大量建筑物:基坑南边有某在建1号楼、某4层砖混2号楼;基坑西边有某新开发项目和某8层钢筋混凝土 3号楼;基坑西北角有某4层砖结 构4号楼;基坑东北角有某4层砖结构的5号楼;基 坑东边有某4层砖结构6号楼、某4层砖结构7号 楼及某5层砖结构8号楼.除1号楼外,其余的邻近 建筑物均有50至1(0年的历史.在基坑开挖之前,在新开发项目和基坑之间施工了厚1m、深30 m的地下连续墙.基坑西边的8层钢筋混凝土结构采用预应力高强度混凝土管桩深基础支撑,其余建筑物 均采用条形基础,属于典型的浅基础建筑物.基坑周 边还有大量地下管线设施:条混凝土雨水管道、2条铸铁供水管道、2条铸铁天然气供应管道、1条铸 铁通讯电缆管道、3条铸铁电力管道及其他电力管道.这些管道的埋置深度为地表以下0. 50〜1m.2地质条件根据地质勘探报告,地表以下2m为填土层、地表以下2〜7 m为粉质黏土层、地表以下7〜18 m 为淤泥质黏土层、地表以下18〜39 m为粉质黏土 层、地表以下39〜43 m为黏土层、地表以下43〜56 m为密实粉砂层、地表以下56〜70 m为密实粉砂 夹砂质黏土层,各土层的物理力学性质参数见图2,其中,^为土层深度、y为土体重度、c’为有效黏聚力为有效内摩擦角、艮为压缩模量、e为孔隙比、为灵敏度、Su为不排水抗剪强度.长期观测的地下水位线为地表以下0.5〜0.7 m .第8期康志军,等.上海软土地区某逆作法地铁深基坑变形[]浙江大学学报:工学版,2017,51(8): 1527 1536.15293围护结构设计方案本基坑采取逆作法施工,支护结构采取“地下连续墙+混凝土支撑+钢支撑”的形式.本工程采用 1 200、1 000、800 m m 这3种规格的地下连续墙,南 端头井地下连续墙深55 m ,北端头井地下连续墙深 46 m ,中间标准段地下连续墙深44 m .南端头井幵 挖深度为26. 1 m ,共设7道支撑,第1、道为混凝 土支撑,其余为钢支撑,下一层板框架逆作法施工; 北端头井幵挖深度为25. 8 m .共设7道支撑,第1、 道为混凝土支撑,其余为钢支撑,下一层板框架逆作法施工;中间标准段幵挖深度为24. 2 m ,共设7道支撑,第1、3、5道为混凝土支撑,其余为钢支撑,下 一层板逆作法施工.车站主体结构基础底部标准段 每隔3 m 抽条加固,加固深度为坑底以下3 m ,其中 封堵墙以北部分标准段第6道支撑底2. 5 m 范围内 及坑底以下3 m 范围内进行旋喷桩加固;南端头井 第3、道支撑底2.5 m 范围内及坑底以下3 m 范围 内旋喷桩加固;北端头井第6道支撑底2. 5 m 范围 内及坑底以下3 m 范围内旋喷桩加固,要求加固土 体28 d 无侧限抗压强度gu >1. 2 M P a .如图3所示为中间标准段支护结构剖面.图1基坑平面及测点布置图F ig. 1S ite p la n o f p ro je c t a lo n g w ith in s tru m e n ta tio n s la y o u t7/(kN • m'3) cVkPa 15 20 25 0 102030405060Su /kPa 0 20 40 60 80(p'Kl EJ MPa e St0 10 20 30 40 0 5 10 15 20 0.00.5 1.01.5 2.0 0 1 2 3 4 5■填土昆粉质勃土层淤泥质勃土层—最小值 —最夫i 直12025 a 3〇 ^ 35 40 45 50606570I-平均值F ig. 2 S o il p ro file s and m a in p h y s ic -m e c h a n ic a l p a ra m e ters1530浙江大学学报(工学版)第51卷图4开挖深度与围护结构最大侧移关系F ig. 4R e la tio n s h ip s b e tw e e n m a x im u m w a ll d e fle c tio n s c ^h m and e xc a v a tio n d e p th H图3中间标准段支护结构剖面F ig. 3P ro file o f s u p p o rtin g s tru c tu re s a t c e n tra l s ta n d a rd segm ents4基坑监测方案为全面掌握施工中基坑变形及对周边环境的影响,对该基坑从以下几个方面进行了动态监控:地下 连续墙侧向变形、墙顶位移、支撑轴力、地下水位、立 柱隆起、周边地表沉降、周围建筑物沉降、管线沉降, 测点布置如图1所示.图1中仅列出雨水管线的测 点分布图,其余管线的走向和测点分布与雨水管线 类似,不再单独列出.5 施工工况基于缩短施工周期、减少基坑幵挖对周围环境的影响等因素.本基坑采取分区段幵挖,按南端头井 —北端头井—换乘大厅中间标准段的先后顺序施 工,各部分的施工周期见表1表中z 为持续时间.开挖区段开始开挖开挖结束t/d南端头井2007-11-292008-4-8130北端头井2008-3-22008-7-14137中间标准段2008-8-262008-10-2561本基坑采取逆作法施工,中间标准段的主要施 工工况及持续时间如表2所示.值得注意的是本基 坑采取移动钢支撑的设计方案:即在幵挖至深度5 和深度8时分别将原本安装于深度4和深度7的钢 支撑移至相应深度,具体施工工况参见图3和表2.6监测数据分析6.1围护结构侧移如图4所示为基坑幵挖至不同深度时,各测斜点处围护结构最大侧移C h m 与基坑幵挖深度H 的关 系.从图中可知,中间标准段的围护结构最大侧移普 遍较大:中间标准段C hm 的变化范围为(0. 25%〜 0.45%)H ,明显大于端头井C hm 的变化范围(0. 10%〜 0.25%)H .这是由于端头井的空间角效应显著,在 一定程度上限制了围护结构侧向变形的发展[5,16].如图5所示为幵挖至坑底时Q 9和Q 10测点处围护结构侧向位移曲线,C 为围护结构侧向位移,z 为围护结构深度.从图1可知Q 9与Q 10测点均位 于中间标准段跨中、且对称布置,但Q 9测点的侧向 位移明显大于Q 10测点.这是由于Q 10测点位于基 坑西侧,邻近的已建地下连续墙和两层换乘大厅等 既有地下结构对基坑变形有显著的遮拦作用,从而 限制了 Q 10测点处围护结构的侧向变形,这与T a n表 13个区段的施工持续时间T a b. 1C o n s tru c tio n d u ra tio n o f 3 se ctions第8期康志军,等.上海软土地区某逆作法地铁深基坑变形[]浙江大学学报:工学版,2017,51(8):1527 1536.1531表2中间标准段主要施工工况T a b. 2 M a in stages o f c o n s tru c tio n a t c e n tra l sta n d a rd segm ents工况施工内容起止时间t/d S l(a)施工地下连续墙、粧基施工2006-12-18〜2007-10-4290 S l(b)注浆加固土体2008-7-31〜2008-8-1617 S2(a)开挖至1.50m(深度1)2008-8-261 S2(b)浇筑混凝土支撑(1. 50 m X0.40 m)2008-9-27〜2008-8-282 S2(c)养护混凝土支撑(深度1)2008-8-29〜2008-9-57 S3 (a)开挖至6. 22 m(深度2)2008-9-11〜2008-9-133 S3(b)安装钢支撑(深度2、妁09 m m)2008-9-131 S3(c)浇筑混凝土顶板(0. 80 m厚)2008-9-14〜2008-9-152 S3(d)养护混凝土顶板2008-9-16〜2008-9-238 S4(a)开挖至10. 22 m(深度4)2008-9-24〜2008-9-263 S4(b)安装钢支撑(深度4、彡609 m m)2008-9-261 S4(c)浇筑混凝土支撑(1 m X 0. 80 m)和楼板1(0. 40 m厚)2008-9-27〜2008-9-293 S4(d)养护混凝土支撑和楼板1(深度3)2008-9-30〜2008-10-67 S5 (a)开挖至12.82m(深度5)2008-10-7〜2008-10-93 S5(b)移动深度4的钢支撑至深度52008-10-91 S6(a)开挖至17.17m(深度7)2008-10-10〜2008-10-123 S6(b)安装钢支撑(糾09 m m、深度7)2008-10-121 S6(c)浇筑混凝土支撑(1. 20 m X0.80 m)和楼板2(0. 40 m厚)2008-10-13〜2008-10-142 S6(d)养护混凝土支撑和楼板2(深度6)2008-10-15 〜2008-10-217 S7(a)开挖至18. 97 m(深度8)2008-10-211 S7(b)移动深度7的钢支撑至深度82008-10-211 S8(a)开挖至21.77m(深度9)2008-10-221 S8(b)安装钢支撑(彡609 m m、深度9)2008-10-221 S9开挖至24. 42 m(最终开挖深度)2008-10-23〜2008-10-253 S10(a)浇筑混凝土底板(1. 30 m厚)2008-10-26〜2008-10-294 S10(b)养护混凝土底板2008-10-29〜2008-12-1952图5开挖至坑底时Q9与Q10侧向变形曲线F ig. 5 F in a l la te ra l d e fle c tio n s o f d ia p h ra g m w a lls a t Q9and Q10等[17]和朱炎兵等[18]针对软土地区顺作法基坑的研究结果相似.6. 2墙顶水平位移如图6所示为中间标准段的围护结构墙顶水平 位移I时程曲线,正值表示墙顶向基坑幵挖侧移动,负值表示墙顶向非幵挖侧移动.从图中可以看到:基坑西侧测点(Q8、Q10、Q12、Q14)的墙顶水平 位移值不超过2m m,且在幵挖过程中保持稳定;而 基坑东侧的测点(Q7、Q9、Q11、Q13)向幵挖一侧产生 较大的水平位移,尤其是当幵挖深度大于17. 17 m 时,东侧的墙顶水平位移明显增大,待底板浇筑后墙 顶水平位移保持稳定.这是由于已建2层换乘大厅的 楼板结构与基坑西侧的围护结构联结成一整体,有效 地限制了相应位置处围护结构的墙顶水平位移.如图7所示为中间标准段东侧的围护结构最大 侧移时程曲线.从图中可以看到,始终呈近似1532浙江大学学报(工学版)第51卷图6中间标准段墙顶水平位移时程曲线F ig. 6 D e v e lo p m e n t o f h o riz o n ta l d isp la ce m e n ts a t w a ll to p o f c e n tra l sta n d a rd seg m ents养j 户底板图7中间标准段东侧围护结构最大侧移时程曲线F ig. 7D e v e lo p m e n t o f m a x im u m w a ll d e fle c tio n s ^h m o f e a ste rn c e n tra l sta n d a rd segm ents的线性增长,当幵挖深度大于17. 17 m 时,^m 没有 发生明显的突变,这表明当幵挖深度大于17. 17 m 时,东侧的围护结构并未产生向基坑幵挖侧的整体 水平位移突变,仅有墙顶产生向基坑幵挖侧的水平 位移突变结合图6、分析导致中间标准段东侧墙顶水平 位移在幵挖深度大于17. 17 m 时发生明显突变的 原因可能是运输车辆等临时地面超载所引起.6.3立柱隆起如图8所示为中间标准段的立柱隆起“时程 曲线.从图中可以看到:立柱隆起在基坑幵挖初期呈 线性增长、在养护混凝土楼板1阶段保持相对稳定、 当基坑幵挖至17. 17 m 后保持较高速率的增长、底 板饶筑后略有回洛并保持稳定.总体来说,位于端部图8中间标准段立柱隆起时程曲线F ig. 8D e v e lo p m e n t o f v e rtic a l disp la ce m e n ts o f in te r io r c o lu m n s a t c e n tra l sta n d a rd segm ents的立柱隆起量(L 17、L 18、L 25)小于中部的立柱 (乙19丄20山21丄22),这符合文献[5,9,15]关于坑 底土体回弹呈“中间大、两端小”分布的结论;此外, 两端端头井已建地下结构亦会抑制端部的立柱隆起. 同一监测断面的立柱隆起量有明显的差异,如L 17和L 18测点的最大隆起量相差20 m m ,这可能是立柱与混凝土楼板结构联结强度的差异性导致的.如图9所示为基坑幵挖深度与立柱隆起的关 系.从图中可以看到:中间标准段立柱隆起L v 的变 化范围较比端头井大,这是由于其幵挖跨度较大导 致.中间标准段L v 的上限为0. 2 6 % H ,明显大于端 头井L的上限0. 18%H ,这是由于端头井的空间角效应显著[5],限制了坑底土体回弹,导致立柱的隆 起量较小.60 50 40 ^ 3020 10►中央标准段/、端头井d-(1)L V =0.26%//,(2)L V =0.18%//®4,!十 ^(2)10 1520 25 30Him图9开挖深度与立柱隆起关系F ig. 9 R e la tio n s h ip s b e tw e e n c o lu m n u p lifts L v andca v a tio n d e p th Hs /I I /i z<n/i i /800<nW I/I I /i <N 0I /I I /i<N5/i i /800<n I e /O I i o<N9<n/0i /800<n +i<n /o i /800<n ■::9I /0I /800<N 曰 4I l /O I i s9/0i /800<n i /o i/800<n9<n /6/800<n I r N I l 9i /6/800<n311191-M 4y 800<N 2 -挖开012 3 4C---*00111111 11 11Q QQ Q Q Q Q Q i £/o i/800<n9<n/0i /800<ni<n/o i /800<n9i /0i /800<ni i /o i/800<n9/0i /800<ni /o i/800<nB ffl 9<n/6/800<ni<n/6/800<n 9i /6/800<ni i /6/800<n9/6/800<n>i /6/800<n第8期康志军,等.上海软土地区某逆作法地铁深基坑变形[]浙江大学学报:工学版,2017,51(8):1527 1536.15336.支撑轴力如图10所示为Z3测点的支撑实测轴力F的时程曲线,正值表示压力.Z3-1至Z3-7的设计轴力 值F。
沉降处理的参考文献
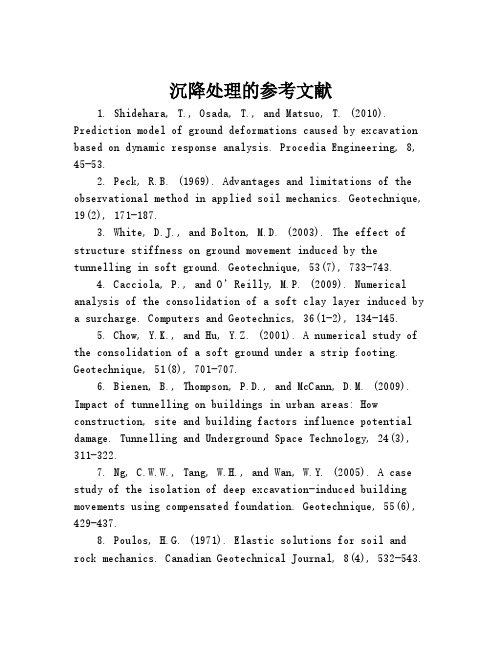
沉降处理的参考文献1. Shidehara, T., Osada, T., and Matsuo, T. (2010). Prediction model of ground deformations caused by excavation based on dynamic response analysis. Procedia Engineering, 8, 45-53.2. Peck, R.B. (1969). Advantages and limitations of the observational method in applied soil mechanics. Geotechnique, 19(2), 171-187.3. White, D.J., and Bolton, M.D. (2003). The effect of structure stiffness on ground movement induced by the tunnelling in soft ground. Geotechnique, 53(7), 733-743.4. Cacciola, P., and O’Reilly, M.P. (2009). Numerical analysis of the consolidation of a soft clay layer induced bya surcharge. Computers and Geotechnics, 36(1-2), 134-145.5. Chow, Y.K., and Hu, Y.Z. (2001). A numerical study of the consolidation of a soft ground under a strip footing. Geotechnique, 51(8), 701-707.6. Bienen, B., Thompson, P.D., and McCann, D.M. (2009). Impact of tunnelling on buildings in urban areas: How construction, site and building factors influence potential damage. Tunnelling and Underground Space Technology, 24(3), 311-322.7. Ng, C.W.W., Tang, W.H., and Wan, W.Y. (2005). A case study of the isolation of deep excavation-induced building movements using compensated foundation. Geotechnique, 55(6), 429-437.8. Poulos, H.G. (1971). Elastic solutions for soil and rock mechanics. Canadian Geotechnical Journal, 8(4), 532-543.9. Mitwally, H. (2012). Numerical simulation of the consolidation problem caused by deep excavations in clay deposits. International Journal of Advanced Structural Engineering, 4(3), 213-223.10. Peuchen, J., and Dias, D. (2016). Monitoring ofground deformations induced by tunnel excavation. Tunnelling and Underground Space Technology, 59, 40-50.以上是一些关于沉降处理的参考文献,这些文献涵盖了地质构造、地下挖掘、软土层固结、建筑物振动等方面的研究。
干旱形成机制与预测理论方法及其灾害风险特征研究进展与展望

doi:10.11676/qxxb2024.20230095气象学报干旱形成机制与预测理论方法及其灾害风险特征研究进展与展望*张 强1,2 李栋梁3 姚玉璧4 王芝兰1 王 莺1 王 静1 王劲松1 王素萍1 岳 平1 王 慧3 韩兰英5 司 东6 李清泉7 曾 刚3 王 欢8ZHANG Qiang1,2 LI Dongliang3 YAO Yubi4 WANG Zhilan1 WANG Ying1 WANG Jing1 WANG Jinsong1 WANG Suping1 YUE Ping1 WANG Hui3 HAN Lanying5 SI Dong6 LI Qingquan7ZENG Gang3 WANG Huan81. 中国气象局兰州干旱气象研究所/甘肃省干旱气候变化与减灾重点实验室/中国气象局干旱气候变化与减灾重点开放实验室,兰州,7300202. 甘肃省气象局,兰州,7300203. 南京信息工程大学大气科学学院,南京,2100444. 兰州资源环境职业技术大学气象学院,兰州,7300215. 兰州区域气候中心,兰州,7300206. 中国科学院大气物理研究所,北京,1000297. 国家气候中心,北京,1000818. 四川师范大学地理与资源科学学院,成都,6100661. Lanzhou Institute of Arid Meteorology,China Meteorological Administration/Key Laboratory of Arid Climate Change and Reducing Disaster of Gansu Province/Key Open Laboratory of Arid Climate Change and Reducing Disaster,Lanzhou 730020,China2. Gansu Meteorological Bureau,Lanzhou 730020,China3. School of Atmospheric Sciences,Nanjing University of Information Science and Technology,Nanjing 210044,China4. School of Meteorology,Lanzhou Resources & Environment Voc-Tech University,Lanzhou 730021,China5. Lanzhou Regional Climate Center,Lanzhou 730020,China6. Institute of Atmospheric Physics,Chinese Academy of Sciences,Beijing 100029,China7. National Climate Centre,China Meteorological Administration,Beijing 100081,China8. School of Geography and Resource Sciences,Sichuan Normal University,Chengdu 610066,China2023-06-25收稿,2023-09-14改回.张强,李栋梁,姚玉璧,王芝兰,王莺,王静,王劲松,王素萍,岳平,王慧,韩兰英,司东,李清泉,曾刚,王欢. 2024. 干旱形成机制与预测理论方法及其灾害风险特征研究进展与展望. 气象学报,82(1):1-21Zhang Qiang, Li Dongliang, Yao Yubi, Wang Zhilan, Wang Ying, Wang Jing, Wang Jinsong, Wang Suping, Yue Ping, Wang Hui, Han Lanying, Si Dong, Li Qingquan, Zeng Gang, Wang Huan. 2024. Progress and prospect of the research on drought formation, prediction, and related risk assessment. Acta Meteorologica Sinica, 82(1):1-21Abstract Under the background of climate warming, the frequency and intensity of droughts are increasing. The regularity of drought occurrence and the complexity of its formation mechanism are becoming more prominent, which poses new challenges to the mechanism study on drought formation, the theory and method of drought prediction and changes in disaster risk. They also restrict* 资助课题:国家自然科学基金重点项目(42230611)。
Ground Movement Predictions for Braced Excavations

Hale Waihona Puke of nonlinear soil stiffness are well understood and have been incorporated into numerical models, many of these models are relatively complex and the parameters lack clear physical meaning. Also, these analyses require special soil testing and lengthy computer calculations and therefore occupy a disproportionate time for practicing engineers. This may explain why engineers generally prefer to use design charts which incorporate nondimensional soil strength and stiffness ratios based on rather simple characterizations ͑Mana and Clough 1981; Clough et al. 1989͒.
DOI: 10.1061/͑ASCE͒1090-0241͑2006͒132:4͑465͒
Resonance structure in the Li^- photodetachment cross section
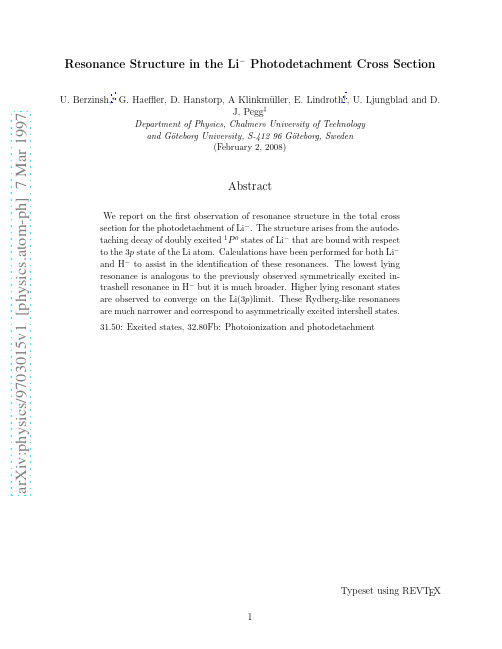
arXiv:physics/9703015v1 [physics.atom-ph] 7 Mar 1997
Resonance Structure in the Li− Photodetachment Cross Section
U. Berzinsh,∗ G. Haeffler, D. Hanstorp, A Klinkm¨ uller, E. Lindroth† , U. Ljungblad and D. J. Pegg‡
2
FIGURES
Ion beam
+ + -
Faraday Neutral particle cup detector
+ +
e Glass QD QD plate CEM Laser beam FIG. 1. Portion of the collinear laser ion beam apparatus shБайду номын сангаасwing the interaction and detection regions. Two quadrupole deflectors (Q.D.) are used to merge the two beams.
Typeset using REVTEX 1
Processes such as double excitation and ionization of few-electron atomic systems provide valuable in
Fluid-Structure Interaction and Dynamics

Fluid-Structure Interaction and Dynamics Fluid-structure interaction (FSI) and dynamics is a complex and multifaceted field that encompasses the study of the interaction between fluid flow and solid structures. This interaction can occur in a wide range of natural and engineered systems, including but not limited to aircraft wings, wind turbines, blood flow in arteries, and ocean structures. Understanding and accurately predicting the behavior of these systems is crucial for the design and optimization of various engineering applications, as well as for the advancement of scientific knowledgein fields such as biomechanics and environmental fluid dynamics. One of the key challenges in FSI and dynamics is the development of accurate and efficient computational models that can capture the intricate interplay between fluid and solid mechanics. The inherent complexity of FSI problems often requires the use of advanced numerical methods, such as finite element analysis, computational fluid dynamics, and immersed boundary methods. These methods must be able to handle the nonlinear and coupled nature of FSI phenomena, while also accounting for factors such as turbulence, fluid-structure coupling, and large deformations of the solid structure. From a practical standpoint, FSI and dynamics play a critical role in the design and performance evaluation of engineering systems. For example, in the aerospace industry, FSI simulations are used to assess the aerodynamic performance and structural integrity of aircraft components, such as wings and control surfaces, under various flight conditions. Similarly, in the field of civil engineering, FSI analysis is employed to study the effects of fluid-structure interaction on the stability and safety of offshore platforms, bridges, and dams. In the biomedical field, FSI models are utilized to investigate the behavior of blood flow in arteries and the impact of cardiovascular diseases. Moreover, FSI and dynamics research has significant implications for the development of medical devices and treatments. For instance, the design of artificial heart valves and stents relies on accurate predictions of the fluid-structure interaction withinthe cardiovascular system. By gaining a deeper understanding of these interactions, researchers and engineers can improve the performance and longevity of such devices, ultimately benefiting the health and well-being of patients with cardiovascular conditions. Despite the progress made in FSI and dynamics, thereare still numerous open questions and research challenges that require further exploration. For instance, the accurate modeling of fluid-structure coupling at different length and time scales remains a daunting task, particularly in the context of biological systems and microfluidic devices. Additionally, the development of robust and efficient numerical algorithms for FSI simulations is an ongoing area of research, as computational costs and convergence issues can pose significant obstacles, especially for large-scale and transient problems. In conclusion, fluid-structure interaction and dynamics represent a fascinating and vital area of study with far-reaching implications for engineering, science, and medicine. The intricate interplay between fluid flow and solid structures presents numerous challenges and opportunities for innovation, ranging from the development of advanced computational methods to the design of novel engineering solutions. As researchers continue to push the boundaries of FSI and dynamics, it is clear that the insights gained from this field will continue to shape our understanding of complex systems and drive technological advancements across diverse disciplines.。
煤矿开采影响地表横向剪切变形论文中英文资料对照外文翻译文献综述
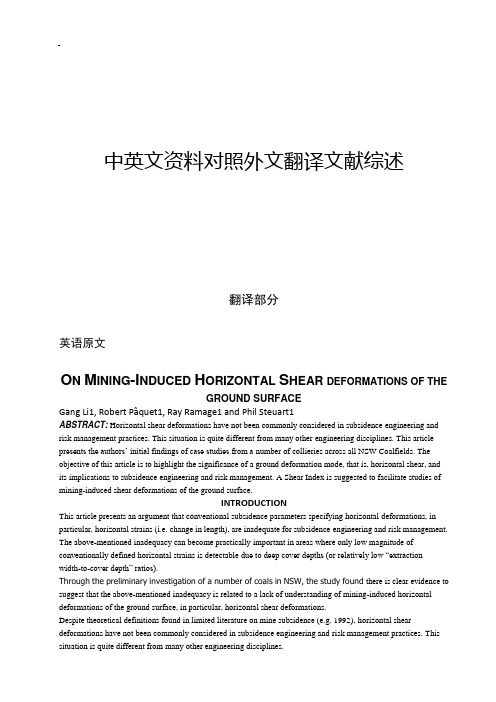
中英文资料对照外文翻译文献综述翻译部分英语原文O N M INING-I NDUCED H ORIZONTAL S HEAR DEFORMATIONS OF THEGROUND SURFACEGang Li1, Robert Pâquet1, Ray Ramage1 and Phil Steuart1ABSTRACT:Horizontal shear deformations have not been commonly considered in subsidence engineering and risk management practices. This situation is quite different from many other engineering disciplines. This article presents the authors’ initial findings of case studies from a number of collieries across all NSW Coalfields. The objective of this article is to highlight the significance of a ground deformation mode, that is, horizontal shear, and its implications to subsidence engineering and risk management. A Shear Index is suggested to facilitate studies of mining-induced shear deformations of the ground surface.INTRODUCTIONThis article presents an argument that conventional subsidence parameters specifying horizontal deformations, in particular, horizontal strains (i.e. change in length), are inadequate for subsidence engineering and risk management. The above-mentioned inadequacy can become practically important in areas where only low magnitude of conventionally defined horizontal strains is detectable due to deep cover depths (or relatively low “extractionwidth-to-cover depth” ratios).Through the preliminary investigation of a number of coals in NSW, the study found there is clear evidence to suggest that the above-mentioned inadequacy is related to a lack of understanding of mining-induced horizontal deformations of the ground surface, in particular, horizontal shear deformations.Despite theoretical definitions found in limited literature on mine subsidence (e.g. 1992), horizontal shear deformations have not been commonly considered in subsidence engineering and risk management practices. This situation is quite different from many other engineering disciplines.HORIZONTAL SHEAR DEFORMATIONSWhen two adjacent cross sections of a stem has a pair of horizontal force perpendicular to stem axis but works in the opposite direction of breaking, and it produces deformation that two section along the lateral force direction of relative rupture occurred. The deformation called shear deformation.Indicators of horizontal shear deformations, as identified by this study, comprise:1.Observed subsidence effects on civil structures indicating influence of shear deformations and significance ofthis deformation mode in terms of its impacts and frequency of occurrences.The shear effects at a particular site are demonstrated in Figure 1;2. S tatistical information suggesting a strong correlation between the shear -affected structures and strip footings, which have less capacity to resist or accommodate horizontal shear deformations as compared with that for other types of footings considered in this study.The analyses show that the transverse shear deformation effect has a significant influence on the thick reinforced concrete slabs and the concentrated load condition;3. Observed patterns of mining-induced surface fractures and deformations (in plan view) suggesting influence of shear, for example, i) en-echelon fractures near chain pillars where shear deformations were active or ii) occurrences of surface wrinkles where the effects of horizontal shear were clearly visible4. Importantly, horizontal shear deformations of ground surface as indicated in 3D survey data obtained from a number of collieries across all NSW Coalfields (to be further discussed).However, rigorous definition, in accordance with the principles of continuum mechanics (e.g. Jaeger, 1969), of horizontal shear strains is not possible using 3D survey data from a straight line of survey points.It follows that if warranted considering the significance of the surface features and their capacity to resist or accommodate shear deformations, the current surveying practices may need to be changed to obtain properly defined horizontal shear strains (or principal strains). To utilise the large amount of subsidence data in existence in the mining industry, an alternative (and approximate) Shear Index is suggested in order to gain an understanding of the general characteristics of mining-induced horizontal shear deformations. This Shear Index is derived based on the component of horizontal movements perpendicular to a survey line or a line of interest. The formula for deriving this index is the same as that for the conventionally defined tilt. Physically, this index reflects angular changes in the horizontal plane but it is not possible to tell what causes such changes, being either shear or rigid body rotation or both. However, the distribution pattern of this index can help to understand the development of shear deformations and to find "trouble spots" (refer to further discussions presented in the Section below).FURTHER DISCUSSIONS ON HORIZONTAL SHEAR DEFORMATIONSFigure 2 shows the distribution pattern of horizontal movements perpendicular to a survey line across a longwall panel and the corresponding Shear Index as discussed above.Although the site is located in the Hunter Coalfield with shallow cover depths, this case is selected as it provides a clear demonstration of the following observations common to the studied cases from all NSW Coalfields:•A complex history of the horizontal movements perpendicular to the cross line (Figure 2a) involving a reversal ofmovement direction after the extraction face passed the survey site by a certain distance. This distance varied from site to site. Similar findings were reported by Holla and Thompson (1992) and Mills (2001);•Indications of horizontal shear deformations (near both solid ribs in this case, as shown by the Shear Index plottedin Figure 2b), noting the reversal in the sense of shearing after the extraction face has passed the survey site. The reversal in the sense of shearing has a potential to enhance the effects of shear deformations, and•The occurrences of permanent horizontal deformations.IMPLICATIONSFrom the 3D survey data collected from a number of collieries across all NSW Coalfields, the characteristics (i.e. the magnitude, nature, distribution and timing of occurrences) of the conventionally defined subsidence parameters are compared with those of the following horizontal deformational parameters:(i) Mining-induced horizontal movements perpendicular to survey grid lines, and(ii) The corresponding Shear Index as discussed above.Implications from the findings of the current study so far are summarised as follows.1.Horizontal Shear Deformations – There is a need to recognise horizontal shear deformation as a significantmode of mining-induced deformations at the ground surface. Specific attention should be paid to surfacefeatures with inadequate shear resistance and to areas with deep cover depths (or relatively low “extraction width-to-cover depth” ratios) where the conventionally defined horizontal strains predicted may suggest low risks.2. Assessment of Subsidence Impacts on Civil Structure s – Further to Point (1) above, there is a need to recognise the limitations of subsidence models based on conventionally defined horizontal strains and AS 2870-1996 (Standards Australia, 1996) when predicting subsidence impacts on civil structures. Consequently, there is a need to identify areas where changes and improvements to these models are required.3. Civil Structures on Sloping Ground – Further to Point (1) above, specific attention should be paid to civil structures on the sloping ground. In this case, there is a potential for enhanced shear deformations due to the participation of down-slope movements. In addition, the performance of any footings to resist or accommodate shear deformations in this environment needs to be investigated and understood.4. Capacity of Surface Features to Resist or Accommodate Shear Deformations – This is an area where knowledge has not been clearly established for subsidence engineering and management. The situation here, again, is different from many other engineering disciplines when shear deformations are concerned. There is a need to undertake necessary research into this area.5. Mining-induced Surface Wrinkles – Mining-induced surface wrinkles (Figure 3), or compression humps, are one of the significant factors for subsidence impacts on civil structures. Where these deformational features occurred in areas with low predicted horizontal strains according to conventional subsidence models, geological structures were often blamed for their occurrences resulting in unpredicted or higher-than-predicted impacts on civil structures. However, recently conducted field investigations have not been able to provide a clear link between geological structures and such surface wrinkles, while there is a continuing need for an improved understanding of these features to develop effective early warning and risk management systems. The identification of horizontal shear deformations can offer an explanation (Figure 4), additional to geological structures and the conventionally defined compressive horizontal strains, for the occurrences of these deformational features.6. Management of Subsidence-related Risks to Linear Infrastructure Items – The results of this study suggest a need to review the adequacy of risk management systems for important linear infrastructure items such as roads, rails, canals or pipelines, if these management systems have been developed based primarily on conventional subsidence models taking into consideration parameters predicted or measured along the lengths of such infrastructure items and/or if the features in questions do not have sufficient capacity to resist or accommodate lateral movements or shear deformations.7. Survey Practices - As discussed above, to obtain properly defined shear strains or principal strains, the survey practices need to be changed. The suggested change is related primarily to the layout of survey grids, for example, 3D surveys of two (or multiple) parallel grid lines separated by an appropriately defined distance.SUMMARYBased on the investigation of the NSW coalfield measurement, this paper analysis the horizontal shear deformation on civil structure influence. This paper research the application of the horizontal shear deformation in the subsidence engineering and risk management system. Finally, the author put forward concerning the horizontal shear deformation field research direction and the prospect of certain.ACKNOWLEDGEMENTThe assistance by NSW Mine Subsidence Board with field investigations and data analysis in relation to civil structures is specifically acknowledged. This article is published with the permission of the NSW Department of Primary Industries. The views expressed in this article are those of the authors.REFERENCESHolla, L and Thompson, K, 1992. A study of ground movement in three orthogonal directions due to shallowmulti-seam longwall mining, The Australian Coal Journal, No.38, pp3-13.Jaeger, J C, 1969. Elasticity, Fracture and Flow with Engineering and Geological Applications, pp268 (Chapman and Hall Science Paperbacks).Mills, K W, 2001. Observations of horizontal subsidence movement at Baal Bone Colliery, inProceedings 5th Triennial Conference on Coal Mine Subsidence Current Practice and Issues, pp 99-111.Peng, S S, 1992. Surface Subsidence Engineering, pp162 (Society for Mining, Metallurgy, andExploration, Inc, Littleton, Colorado).Ramsay, J G, 1980. Shear zone geometry: a review. J. Struct. Geol., Vol. 2, pp83-99 Standards Australia, 1996. Residential Slabs and Footings – Construction (AS 2870-1996).中文译文受开采影响地表横向剪切变形Gang Li1, Robert Pâquet1, Ray Ramage1and Phil Steuart11NSW Department of Primary Industries - Mineral Resources摘要:横向剪切变形尚未普遍应用于沉陷工程风险管理。
基坑监测系统(外文文献) (6)
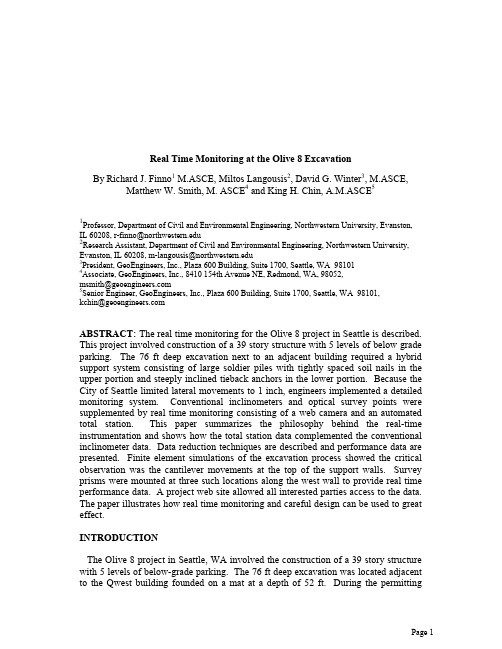
Real Time Monitoring at the Olive 8 Excavation By Richard J. Finno1 M.ASCE, Miltos Langousis2, David G. Winter3, M.ASCE, Matthew W. Smith, M. ASCE4 and King H. Chin, A.M.ASCE5
Py of Seattle stipulated that if the lateral movements were observed to be in excess of ½ inch between two successive readings or if the total wall movements exceeded 1 inch, the construction of the shoring wall would be stopped to evaluate the cause of the movement and to establish the type and extent of remedial measures required. Based on past performance data of excavations through similar soil conditions, typical deflections for excavations of this height (Clough and O’Rourke 1990) were expected to vary from 0.001H to 0.003H, or ¾ inch to about 2.5 inches. Therefore particular attention was paid to designing the support system to limit the movements to less than 1 inch. To meet these requirements, the wall adjacent to the Qwest building was designed with a hybrid support system consisting of large soldier piles with tightly spaced soil nails in the upper portion and steeply inclined tieback anchors in the lower portion. To facilitate timely data acquisition and evaluation during construction, a robotic total station autonomously collected 3-dimensional movements of prisms established at the top of the support wall. These data were placed autonomously on a web site that allowed all interested parties access to the information. The total station data were combined with lateral movements behind the support wall measured with conventional inclinometers. Because design calculations suggested the cantilever movements at the top of the wall could likely have been the largest movements that would occur, the combination of the two types of data would allow essentially real time evaluation of the effects of construction on the ground movements. This paper describes the Olive 8 excavation, discusses the design studies used to develop the instrumentation approach, presents the instrumentation and data acquisition systems employed at the site, summarizes the performance of the support system and compares the design predictions with the observed responses. SITE DESCRIPTION The design of the excavation for the 8th and Olive development in Seattle presented a number of challenges due to the presence of the adjacent Qwest building. A 16 ft alley separated the Olive 8 excavation and the Qwest building, immediately to the west. The Qwest building extends approximately 52 ft below grade and is supported on a perimeter strip footing and a mat foundation for the core of the structure. The design bearing pressure of these foundations was 10 ksf. Existing buried power and communications utilities are located in the alley along with five utility vaults. Because of the movement constraints imposed by these conditions, the design of the excavation support for that wall differed from the other three walls. A cross section through the excavation adjacent to the Qwest building is shown in Figure 1. The shoring system for the west support wall consisted of a combination of soldier piles, soil nails and tiedback ground anchors. The soldier piles were W24x162 sections spaced at approximately 8 ft centers. The upper portion of the alley soils was reinforced with a high density soil nail configuration to the elevation where the tieback anchors were installed. Typically, 9 rows of soil nails were installed at 3 ft vertical by 4 ft horizontal spacing. The soil nails were angled 15 degrees below the horizontal
- 1、下载文档前请自行甄别文档内容的完整性,平台不提供额外的编辑、内容补充、找答案等附加服务。
- 2、"仅部分预览"的文档,不可在线预览部分如存在完整性等问题,可反馈申请退款(可完整预览的文档不适用该条件!)。
- 3、如文档侵犯您的权益,请联系客服反馈,我们会尽快为您处理(人工客服工作时间:9:00-18:30)。
Ž.Tunnelling and Underground Space Technology1720023᎐19Predictions of ground deformations in shallow tunnels in clayWei-I.Chou a,Antonio Bobet b,Ua Professional Ser¨ice Industries,Inc.,Indianapolis,IN,USAb Purdue Uni¨ersity,West Lafayette,IN,USAReceived7May2001;received in revised form15October2001;accepted1November2001AbstractTwenty-eight tunnels are used to evaluate predictions from an analytical solution for shallow tunnels in saturated ground.The solution assumes plane strain conditions at any cross-section perpendicular to the tunnel axis and poroelastic behavior of the ground and elastic behavior of the liner.Stresses and deformations are obtained with this method for short-and long-term conditions anywhere in the continuum.Of particular interest to this study are the short-term surface settlements at the ground parisons between predictions and observations from actual tunnels show good agreement,generally within15%Ž.difference.The analyses show that:1most of the ground movements are caused by the gap parameter,which is a measure of the three-dimensional deformations at the tunnel face,the physical gap between the liner and the perimeter of the excavation,Ž.and of the workmanship;2most of the ground deformations take place within a distance of three to four radii around the Ž.tunnel;3the bottom boundary of zero vertical deformation should be placed at a distance of two tunnel diameters below theŽ.tunnel center line,or at the location of a stiff soil layer,whichever comesfirst;4the horizontal movements around the tunnelŽ.are relatively smaller than the vertical movements;and5the analytical solution tends to underpredict the maximum soil deformations and overestimate the settlement trough;however,with an appropriate estimation of the gap parameter and small soil yield,the differences between predictions and observations are small.ᮊ2002Elsevier Science Ltd.All rights reserved.Keywords:Tunnels;Analytical solution;Saturated ground1.IntroductionDue to recent city developments with limited avail-able land to build on,more and more public facilities are developed under the ground plex un-derground constructions may cause serious damage to existing structures if predictions do not forecast the ground behavior during excavation.Surface settlements are often estimated using empirical methods such asŽ. the Schmidt᎐Peck method Peck,1969;Schmidt,1969, where it is generally assumed that the surface settle-U Corresponding author.Tel.:q1-765-494-5033;fax:q1-765-496-1364.Ž.E-mail address:bobet@ A.Bobet.ment trough can be approximated by the normal probability curve or error function:x2Ž. S s S exp y1 max2ž/2iIn the equation,S is settlement,S is the maxi-maxmum settlement above the tunnel centerline,‘x’is the horizontal distance from the tunnel centerline in the transverse direction,and‘i’is the distance from the tunnel centerline to the inflexion point of the curve. Other empirical or quasi-empirical methods haveŽbeen proposed recently Verruijt and Booker,1996;.Loganathan and Poulos,1998that show good correla-tions between observations from actual tunnels and predictions.Empirical methods,however,have signifi-0886-7798r02r$-see front matterᮊ2002Elsevier Science Ltd.All rights reserved.Ž.PII:S0886-77980100068-2()W.Chou,A.Bobet r Tunnelling and Underground Space Technology 1720023᎐194Ž.cant shortcomings:1they have been developed or Ž.have been validated from a limited number of cases;2they should be applied only to tunnels that fall within the scope of the cases from which the method was Ž.developed;3only few soil and geometry parameters Ž.are taken into account;4they do not consider con-Ž.struction methods;and 5they cannot give the com-plete solution of a tunnel with support.Empirical methods are still widely used;however,predictions of ground movements based on such meth-ods are insufficient for most practical applications.There are a limited number of analytical and numerical tools that can be used to predict ground deformations,but there is a growing demand for developing practical rational methods for tunnel design.This paper focuses on the applicability of one of the recently proposed Ž.rational methods Bobet,2001for shallow tunnels in saturated ground.Although the method is based on elasticity and does not fully account for the behavior of the soil,the predictions are quite good when compared with actual cases,and the solution may be used for preliminary design.2.The analytical solutionThe solution of a shallow tunnel in a saturated Ž.ground has been obtained by Bobet 2001,with the Ž.Ž.following assumptions see Fig.1:a circular cross-Ž.section with radius r ;b plane strain conditions in a o direction perpendicular to the cross-section of the tun-Ž.nel;c frictionless interface between the ground and Ž.Ž.the liner e.g.shield driven tunnels ;d depth to radius Ž.ratio larger than 1.5;e homogeneous and isotropic Ž.ground;f poroelastic behavior of the ground and Ž.Želastic liner;g small thickness of the liner i.e.linerFig.1.Shallow tunnel..Ž.thickness,t <r ;and g permeability of the ground o small enough such that no excess pore pressures dissi-Žpate during construction i.e.undrained conditions ap-.ply .Of particular interest to this study is the short-term Ž.i.e.immediately after construction ground movements of a shallow tunnel in a saturated ground with or without application of air pressure during construction.The following is a summary of the most relevant equa-tions needed for the analysis.Further details can be Ž.found in Bobet 2001.The solution must satisfy the equilibrium equations,the strain compatibility equations,and the boundary conditions.A general solution has been proposed by Ž.Timoshenko and Goodier 1970,in the form of the Airy function,:s a ln r q b r 2q c r 2ln r q d r 2q a Ј0000013y 1Ž.q a r sin q b r q a Јr q b Јr ln r cos 1111213y 1Ž.y c r cos q d r q c Јr q d Јr ln r sin 11112ϱn n q 2y n y n q 2Ž.q a r q b r q a Јr q b Јr cos n Ýn n n n n s 2ϱn n q 2y n y n q 2Ž.qc r qd r q c Јr q d Јr sin n Ýn n n n n s 2Ž.2where the parameters a ,b ,c ,etc.,are determined 000from the boundary conditions.The complete solution is obtained by decoupling the liner and the ground and imposing compatibility of deformations and stresses at the interface between the ground and the liner.The following boundary conditions apply:<<s U s 0s "r 2s "r 2r ground <liner <U s U y w r s r r s r r r o o ground <liner <Ž.s 3r s r r s r r r o oground <liner <Ž.s s 0smooth interface r s r r s r r r o o <Ž.Ž.sy ␥q ␥h y y r ªϱy b w <Ž.s k Јq ␥h y y r ªϱx y w w where x ,y are the Cartesian coordinates with origin atthe center of the tunnel and r ,are the corresponding polar coordinates;,,,U ,U ,are the total r r r stresses and displacements in polar coordinates;,x y()W.Chou,A.Bobet r Tunnelling and Underground Space Technology 1720023᎐195Žare the total stresses in Cartesian coordinates Јis for .effective stresses ;w is the gap parameter,which is the magnitude of the radial soil displacements that occur before the liner provides support;␥and ␥are the b w buoyant unit weight of the ground and the unit weight of the water,respectively;k is the coefficient of earth pressure at rest;h and h are the depth of the tunnel w below the ground surface and below the water table Ž.Ž.Fig.1.Note that the far field stresses i.e.for y ªϱcorrespond to the geostatic stresses in a half-space continuum.Because of the assumption of short-term analysis Ž.i.e.excess pore pressures do not dissipate ,the ground Ž.cannot change volume i.e.s 0.For a poroelastic vol material,this requires:Ž.Јq Јs 04r The stress ᎐displacement relations for the liner are Ž.Flugge,1966:¨2linerlinerŽ2.dU dU C 1y r liner qsyr o r 2d Ed dU liner d 4U liner d 2U linerCr r liner liner q U q q 2q U r r 42ž/d Fd d Ž2.C 1y linerŽ.s r 5o r EC and F are the compressibility and flexibility ratios,respectively,defined as:Ž2.3Ž2.Er 1y Er 1y o s o s Ž.C s ;F s622Ž.Ž.E A 1y E I 1y s s s s where E ,are the Young’s modulus and Poisson’s ratio of the ground;E ,are the Young’s modulus s s and Poisson’s ratio of the liner;A ,I are the area and s s moment of inertia of the cross-section of the liner per Ž.unit length of tunnel Fig.1.2.1.Short-term analysis of a tunnel in saturated ground ()no air pressure Ž.Ž.The solution of Eqs.2᎐6determines the stresses Žand displacements anywhere in the continuum i.e.for .both ground and liner .The ground displacements are of particular interest,and are given by:a c Ј1q 1o 1U sy q q c ln r sin r 12½E r 2r a Јb Јc Јd Ј2233q 2q 2cos2q 3q 3sin33425r r r r c Ј1q 11Ž.U sy y c 1q ln r cos 12½E 2r a Јc Јd Ј233Ž.q 2sin2y 3q cos373425r r r withw Ž.x Ž2.␥h 1q k q 2␥h 1y CFb w w Ž.Ž.q 2E C q F w r r 1o 2a s r 0o22Ž.Ž.Ž.C q F 1q q 1y CFŽ.2c sy ␥q ␥r 1b w o14Ž.c Јsy ␥1y k r 1b o8Ž.11y F q 34Ž.Ž.a Јsy␥h 1y k r 82b o4Ž.1y F q 6Ž.121y F q 32Ž.b Јs␥h 1y k r 2b o4Ž.1y F q 6Ž.11y F q 126Ž.c Јs␥1y k r 3bo12Ž.1y F q 24Ž.11y F q 84Ž.d Јsy␥1y k r 3bo8Ž.1y F q 242.2.Short-term analysis of a tunnel in saturated ground with air pressureThe stresses and displacements of the ground and liner are obtained for the following construction process:1.The tunnel is excavated under air pressure,p .a2.The liner is installed and the air pressure released.During excavation the opening is created and,at the same time,an internal air pressure,p ,is applied.To a prevent water inflow into the tunnel,the value of the air pressure is generally taken as the magnitude of the water pressure at the invert of the tunnel.Ž.Ž.P s ␥h q r 9a w w o Once the excavation is completed,the liner is in-stalled and the air pressure released.At the end of this()W.Chou,A.Bobet r Tunnelling and Underground Space Technology 1720023᎐196Ž.construction process,the solution is given by Eq.7with:122Ž.Ž.a s␥h 1q k r q ␥h y p r 0bo w w a o 2Ž2.Ž.Ž.1y CFp q C q F E w r r a o 2q ro2Ž.Ž.Ž.1q C q F q 1y CF Ž.2c sy ␥q ␥r 1b w o14Ž.c Јsy ␥1y k r 1b o814Ž.Ž.a Јsy ␥h 1y k r 102b o412Ž.b Јs␥h 1y k r 2bo 216Ž.c Јs␥1y k r 3bo 1214Ž.d Јsy ␥1y k r 3b o8Ž.As it can be observed in Eq.7,the analytical solution predicts an increase of displacements with the logarithm of the radial distance.While this is a mathe-Žmatical consequence of the assumptions made i.e.semi-infinite,elastic,isotropic,homogeneous medium,.and infinitely long tunnel ,it has no physical basis.In reality,the soil conditions are not homogeneous and the soil does not extend to an unlimited depth.At a certain distance below the tunnel there will be a stiff Ž.layer e.g.bedrock where the vertical displacements should be zero.In addition,other factors such as an increase of the stiffness of the soil with depth,weight Ž.Žof the Tunnel Boring Machine TBM and liner not .considered in the analytical solution ,and not uniform tail gap around the excavation will reduce at the invert the soil displacements towards the excavation.Hence a correction should be made to the above formulation such as at a certain distance below the tunnel center-line the vertical displacements are zero.Hence,corrected analyticalsolutiontopof stiff layerŽ.U s U y U 11y y y The boundary of zero vertical displacement is chosen Ž.at a depth which is the smallest of:a the depth of Žbedrock or stiff layer a soil layer can be considered stiff when its Young’s modulus is approximately 100times larger than that of the soil where the tunnel is .Ž.excavated ;and b two times the tunnel diameter below the tunnel centerline;this is an empirical ap-proximation obtained from actual case studies and is related to the zone of influence of the excavation.Once this correction is done,the solution recovers physical meaning in that displacements decrease as the distance Ž.from the tunnel increases Bobet,2001.Note that horizontal displacements do not need correction.Ž.Soil displacements,as defined by Eq.7,are very sensitive to the gap parameter,w ,since small variations of w may have a large effect on soil settlements.The gap parameter represents the soil deformations that occur before the soil gets in contact with the liner.Hence,this parameter is a measure not only of the physical gap between the liner and the ground,but also of the soil deformations ahead of the excavation and of Ž.the quality of the workmanship overcutting .The rela-tive importance of this parameter can be evaluated by Žlooking at the maximum settlements at the surface i.e..for s 90Њ,y s h .With typical ground and liner properties the flexibility ratio,F ,is very large.An approximate solution for some tunnels can be obtained Ž.assuming a very flexible F s ϱand incompressible Ž.liner C s 0.If the water table is at the surface,and for a tunnel without air pressure,the maximum settle-Žments,␦,are no corrections for stiff layer are max .used :wr 1q 1o 2␦syq y ␥r ln h max o ½h E 235r r r 31o o oŽ.q ␥h 1y k r y qyb o ž/ž/5h 4h 4h Ž.12For a tunnel excavated under air pressure:wr 1q 1o 2Ž␦syq y ␥r ln h q ␥h 3max o b ½h E 235r r 31oo2.Ž.y k r q ␥h 1y k r yo b ož/ž/4h4h 2r o Ž.q ␥hr 13w ož/5hŽ.Ž.Eqs.12and 13show that for a very flexible and incompressible liner the maximum settlements on the Ž.surface are due to two terms:1a geometric term that Ž.involves the gap parameter;and 2a term that in-volves the ground stiffness,E .The relative importance that each term has on the total settlement depends on the geometry of the tunnel and on the ground stiffness.It is clear that as the stiffness of the ground increases,the gap parameter becomes very important.For other Ž.types of liners i.e.relatively flexible and compressible the stiffness of the liner may have a substantial effect on settlements,particularly the compressibility of the()W.Chou,A.Bobet r Tunnelling and Underground Space Technology1720023᎐197liner since as the parameter C becomes larger theŽsettlements increase note that at the limit,Cªϱ,the.tunnel behaves as if there was no liner.Ž.Ž.A comparison between Eq.12and Eq.13also shows that the ground displacements at the surface are larger for a tunnel excavated under air pressure than without air pressure.This is due to the construction process assumed,where the ground is initially sup-ported only with the air pressure.The definition of the gap parameter necessarily in-troduces some uncertainty on its determination;how-ever,it can be estimated from one of the following two methods:Ž.Ž.1Direct estimation Lee et al.,1992.Ž. w s G q U q workmanship14 p3Dwhere G is the physical gap between the liner and the pperimeter of the excavation and includes the thickness of the TBM tailskin and the clearance required forerection of the liner;U is a measure of the soil3Dmovements ahead of the face of the tunnel;and the workmanship is a measure of the overcutting as theŽTBM is steered Lee et al.,1992,provide details on how to obtain each of the three components of the gap .parameter.Ž.2Back-calculation from the estimated or measured ground loss.22Ž.r q w yr2wo oŽ. Ground loss s(152rr oowhere the ground loss should also include workman-ship.3.Case studiesŽ.A total of14tunnels including28tunnel sections have been investigated.They comprise different con-struction techniques including compressed air,have ground conditions that range from soft to stiff clay,and coefficients of earth pressure at rest ranging from0.5 to1.5.Some of the cases have been investigated by previous researchers and are well documented,and thus they are very well suited to make comparisons with the analytical solution and assess its applicability to actual tunnels.Table1summarizes characteristic soil properties, geometry of the tunnels,and construction methods. Most of the parameters are taken from published re-sults;in a few cases,however,some of the data needed for the analytical solution are not available and have been estimated from other parameters;for example the Poisson’s ratio of the soil,if not available,is taken as 0.2,and the soil Young’s modulus,if not given,is estimated from undrained shear strength S,standardu penetration tests,or from cone penetration tests.If the properties of the liner are not reported in the litera-ture,they are taken as E s25000MPa ands0.3,s s which correspond to a typical support.Table1also gives the stability number N at the face of the excava-tion;the stability number is defined as:␥h yiŽ. N s16 Suwhere␥h is the total vertical stress at the tunnel axis,is the support pressure at the face of the tunnel,and iS is the undrained shear strength of the soil.uFive cases are reviewed in detail in this paper and are used to compare the predictive capabilities of the analytical solution with actual measurements.Three of thefive tunnels are excavated without compressed air, and two with compressed air.The conclusions obtained from the cases presented are thought to be representa-tive and applicable to the rest of the tunnels.3.1.Tunnels without compressed air3.1.1.London Transport Fleet Line,Green Park UndergroundThe Green Park Underground Tunnel was shield driven in stiff heavily overconsolidated London clay. The tunnel was excavated by hand with a 4.146-m diameter shield at a depth of approximately29m.A seven-segment cast-iron lining4.07-m external diame-ter and0.6-m width was erected inside the shield tailskin and water᎐cement grout was injected into the cavity between the liner ring and the excavated soil.A simplified soil profile of the construction site consisted from top to bottom of a layer of sand and gravel down to a depth of2m;underneath there was a very thick layer of stifffissured clay where most of the tunnel was Ž.excavated Attewell and Farmer,1974.The ground-water level was found at a depth of approximately2m. The undrained shear strength of the stiff clay,obtained from unconsolidated undrained triaxial tests,is taken for the analyses as270kPa which is consistent with the reported stability number N of2.2.The elastic modu-Ž. lus of the clay is estimated as50MPa Lo et al.,1984, and the coefficient of lateral earth pressure at rest K0 is1.5,which is typical of London clay.The physical gap between the perimeter of the excavation and the liner is the sum of the size of the bead,6.5mm,and the tail void,76mm.Lo et al.have suggested a gap parameter, w,equal to23mm,based on their evaluation of the physical gap,grouting of the tail void,and face move-()W.Chou,A.Bobet r Tunnelling and Underground Space Technology 1720023᎐199ments;this is the magnitude of the gap parameter taken for the analytical solution.Fig.2shows a comparison between predictions from the analytical solution and observed movements.Fig.3shows observed and predicted soil settlements above the tunnel centerline.The comparisons are quite good.As observed in the figures both the analytical solution and the observed settlements resemble a Gauss probability function.Another interesting observation is that,as shown in Fig.3,most of the soil deformations occur within an area very close to the tunnel,of the order of three to four radii.3.1.2.Bangkok Sewer Tunnel,ThailandThe tunnel is a sewer tunnel built for the Metropoli-tan Water Works Authority in Bangkok.The tunnel was driven at a depth of approximately 18m;it has a total length of 10.5km,and a diameter of 2.67m Ž.Phienwej,1997.Three earth-pressure balanced shields were used to excavate the tunnel and each shield had a diameter of 2.8m and a length of 6.2m.Depending on the ground conditions,the ground control features of the machines ranged from closeable flood door or Ž.stable ground to full earth pressure balance EPB support for unstable ground.The primary support con-sisted of 150-mm-thick and 1-m-long bolted concrete segments with secondary grouting to backfill the annu-lar space behind the segmental rings.Bangkok is situated on a thick series of marine and alluvial soil deposits.Down to 35-m depth the subsoil profile is relatively uniform.A thick soft marineclayFig.2.Green Park Underground Tunnel.Surface settlements.layer with undrained shear strength of 10᎐15kPa and water content 70᎐120%extends from the ground sur-face to a depth of 12᎐15m.Underneath there is a uniform and impervious stiff clay with thickness rang-ing between 5and 15m.A fine to medium dense sand layer appears under the stiff clay with a total thickness ranging between 8and 16m.The undrained Young’s modulus of the stiff clay is approximately 20MPa.The water table is found within the soft marine clay approx-imately 2m below thesurface.Fig.3.Green Park Underground Tunnel.Subsurface settlements above centerline.()W.Chou,A.Bobet r Tunnelling and Underground Space Technology 1720023᎐1910Fig.4.Bangkok Sewer Tunnel.Surface settlements.A gap parameter of 37mm is taken for the analysis,based on the estimated ground loss 3᎐5%and tunnel Ž.geometry Phienwej,1997.The bottom boundary is set at a depth of 23m where a dense sand layer is found.Figs.4᎐6show predicted and observed soil deforma-tions.Fig.4is a plot of surface settlements;Fig.5is a plot of subsurface settlements with depth,and Fig.6shows actual and predicted horizontal displacements at a vertical cross-section perpendicular to the tunnel,4m from the tunnel centerline.Surface settlements and Ž.horizontal displacements Figs.4and 6are predicted reasonably rger differences are observed inFig.Fig.5.Bangkok Sewer Tunnel.Subsurface settlements above centerline.() W.Chou,A.Bobet r Tunnelling and Underground Space Technology1720023᎐1911Fig.6.Bangkok Sewer Tunnel.Subsurface horizontal displacements4m from centerline.5between predictions and measurements,particularly close to the crown of the tunnel.Observed settlements are much larger than predicted settlements;this may be caused by the large deformations that are necessary in the soil tofill the physical gap between the liner and the excavation.Such large deformations are likely to produce plastic deformations in the soil which may account for some of the discrepancies.Another reason for the differences is the layered nature of the soil; although the tunnel is excavated in the stiff clay,there is a thick soft clay deposit near the surface.The influ-ence of this deposit can be noticed on the horizontal displacements depicted in Fig.6,where the slope of the observed settlement curve increases significantly on the top10m of the soil,where the soft deposit is encoun-tered.3.1.3.London Transport Fleet Line,Regent’s ParkTwo tunnels with4.146m diameter were constructed with expanded concrete lining at depths of20.1mŽ.Ž. northbound and34.1m southbound at Regent’s Park,London,as part of the Fleet Line of the LondonŽ. Underground Barratt and Tyler,1976.The vertical distance between the Northbound and the Southbound tunnel centerlines is14m,and the horizontal distance is18m.Both tunnels were shield driven with a3-mm bead and were hand excavated;the southbound tunnel was constructedfirst.Precast concrete segments were used as the primary support and the concrete lining was expanded against the ground immediately after erection.The tunnels were excavated in London Clay,which can be characterized as a stiff,fissured,overconsoli-dated clay.The undrained shear strength of the clay, S,obtained from unconsolidated undrained triaxial utests is230kPa.The Young’s modulus is taken as32 MPa for the northbound tunnel and56MPa for theŽ. southbound tunnel,after Lee et al.1992;the coeffi-cient of lateral earth pressure is1.5,which is typical of London clay.The groundwater table was found at a depth of approximately4m.The ground loss for both tunnels was 1.3᎐1.4%.Ž. Although the tail void was quite small3mm the gap parameter is taken as17mm for the northbound tunnel and23mm for the southbound to account forŽface deformations and workmanship after Lo et al., .1984.Figs.7᎐9show comparisons between the analytical solution and actual measurements.All comparisons are reasonably good.Fig.7is a plot of surface settlements for both the northbound and southbound tunnels.Since the distance between the two tunnels is quite large,it is expected that the construction of thefirst tunnel Ž.southbound should not affect the settlements caused by the construction of the second tunnel;this is shown in thefigure.Fig.8is a plot of the subsurface settle-ments above the centerline of the northbound tunnel. The soil deformations are larger above the crown of the tunnel and decrease as the distance from the tunnel increases;in fact beyond a distance of approxi-mately three tunnel radii the settlements do not in-crease significantly.A similar observation can be made()W.Chou,A.Bobet r Tunnelling and Underground Space Technology 1720023᎐1912Fig.7.Regent Park Underground.Surface settlements.Ž.for the southbound tunnel Fig.9.The settlements of the southbound tunnel above the crown are larger than the northbound tunnel even though the southbound tunnel rests on a relatively stiffer clay compared to the northbound tunnel;this is a result of the larger gap parameter,and for this case is an indication of the relative importance of this parameter compared to the geometry of the tunnel or to the soil properties.3.2.Tunnels with compressed air3.2.1.Belfast Sewer Scheme,Sydenham,BelfastThe tunnel is a sewer tunnel constructed in Belfast;it is 450m long and has a circular cross-section of 2.74m in diameter.The tunnel is rather shallow,with an average depth of 4.85m below the groundsurface.Fig.8.Regent Park Underground.Northbound tunnel.Surface settlements above centerline.Fig.9.Regent Park Underground.Southbound tunnel.Surface settlements above centerline.Considerable settlements were experienced by previ-ous cut and cover excavations.Since the route of the culvert ran close to housing and industrial buildings,it was decided to construct the culvert in the tunnel to reduce surface settlements and minimize damage to the above buildings.The tunnel was shield-driven and was supported by precast concrete rings.The shield had a3-mm bead and a1-m-long tailskin.The rings were composed offive bolted segments0.6m long, which were erected within the tailskin of the shield. Grouting was directly injected into the rear of the tailskin after each shove to reduce settlement.The tunnel was excavated below the groundwater table, which was located at a depth of1.2᎐1.5m below the ground surface.To keep water out of the tunnel and to help stabilize the weak face,the tunnel was constructed2Žwith compressed air at a pressure of48kN r m41 2.kN r m at an earlier stage.This air pressure lowered the stability number N of the face from8.35to3.60. The tunnel was built in an alluvial deposit with sandy layers with variable clay and organic content.Below the surface there was afill deposit between2and3m thick;underneath there was a soft gray silty clay5᎐6m thick followed by a thick stiff brown clay.The tunnel was excavated within the soft silt deposit,under the water table.The silty clay deposit showed highly vari-able engineering properties,with undrained shear strength ranging from8to20.5kN r m2.The gap parameter is obtained from the ground loss,Ž.1.8᎐2.1%,Glossop and Farmer,1977,and ranges between23and28mm.Figs.10and11show predictions and measurementsŽ.at two control locations arrays A and B.As shown in thefigures the predictions are reasonably good.At array A,the settlement trough had a maximum settle-ment of16mm and a width of approximately14m, while at array B the maximum settlement was20mm and the width20m.The difference between the two sites is due to a thicker surfacefill layer at array B,to the variability of the soil properties,and to a different Ž. axial depth 4.55m at array A and4.35m at array B.3.2.2.Central Interceptor Tunnel,Mexico CityThe tunnel was driven with an open face shield6.28 m in diameter and6.37m long at an average depth ofŽ23m through soft clay Schmitter et al.,1981;Schmit-.ter and Rendon,1981.Excavation was full-face hand mined under compressed air.The air pressure applied ranged between127.4and117.6kPa.The air pressure was kept constant within the pressurized chamber until the secondary lining was started.The primary lining consisted offive precast concrete segments,75cm long and25cm thick,which were assembled within the shield.A void of7cm was left between the liner and the excavated soil and wasfilled with pea gravel and grout.There were six instrumented sections to measure soil deformations.Thefirstfive sections were located at depths of approximately23.5m and the sixth section was located at a depth of27m.The soil profile,as an average,consisted of a layer of silty sandy soil and clay down to a depth of5m below the surface.Underneath there was a soft,compressible clay,down to a depth of25m;interspersed within this layer at different depths there were sandy silt and silty。