Optical properties of GaN grown on Si by gas source
硅基与蓝宝石衬底上的GaN-LEDs性能差异分析
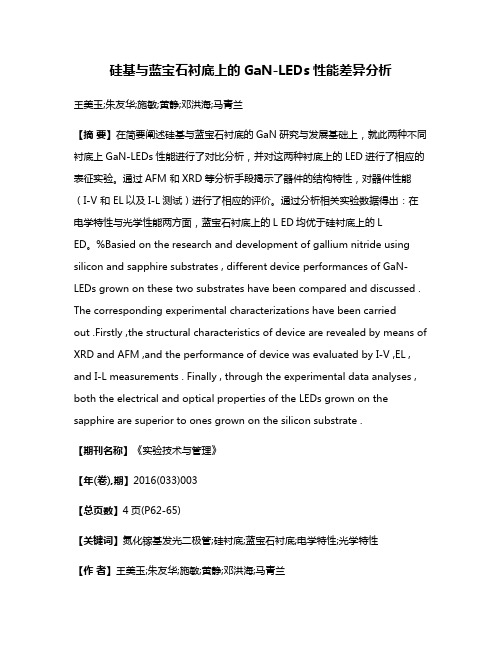
硅基与蓝宝石衬底上的GaN-LEDs性能差异分析王美玉;朱友华;施敏;黄静;邓洪海;马青兰【摘要】在简要阐述硅基与蓝宝石衬底的GaN研究与发展基础上,就此两种不同衬底上GaN‐LEDs性能进行了对比分析,并对这两种衬底上的LED进行了相应的表征实验。
通过AFM 和XRD等分析手段揭示了器件的结构特性,对器件性能(I‐V 和 EL以及I‐L测试)进行了相应的评价。
通过分析相关实验数据得出:在电学特性与光学性能两方面,蓝宝石衬底上的L ED均优于硅衬底上的L ED。
%Basied on the research and development of gallium nitride using silicon and sapphire substrates , different device performances of GaN‐LEDs grown on these two substrates have been compared and discussed . The corresponding experimental characterizations have been carriedout .Firstly ,the structural characteristics of device are revealed by means of XRD and AFM ,and the performance of device was evaluated by I‐V ,EL , and I‐L measurements . Finally , through the experimental data analyses , both the electrical and optical properties of the LEDs grown on the sapphire are superior to ones grown on the silicon substrate .【期刊名称】《实验技术与管理》【年(卷),期】2016(033)003【总页数】4页(P62-65)【关键词】氮化镓基发光二极管;硅衬底;蓝宝石衬底;电学特性;光学特性【作者】王美玉;朱友华;施敏;黄静;邓洪海;马青兰【作者单位】南通大学电子信息学院,江苏南通226019;南通大学电子信息学院,江苏南通 226019;南通大学电子信息学院,江苏南通 226019;南通大学电子信息学院,江苏南通 226019;南通大学电子信息学院,江苏南通 226019;南通大学电子信息学院,江苏南通 226019【正文语种】中文【中图分类】TN364近年来,新型宽禁带化合物半导体材料的发展迅速,特别是GaN材料。
氮化镓纳米粒子的制备及光致发光研究

氮化镓纳米粒子的制备及光致发光研究沈龙海;富松;石广立【摘要】采用直接氮化法制备出尺寸不同的GaN纳米粒子,分别利用X射线衍射仪(XRD)、扫描电子显微镜(SEM)和光致发光(PL)谱测试手段对所制样品进行表征和发光特性的研究.结果表明:所制备的两种GaN纳米粒子直径分别为100 nm和300 nm左右.在GaN纳米粒子的PL谱中,中心在357 nm的发射源于本征发光,中心在385 nm的发射带源于浅施主能级到价带的辐射复合,中心在560 nm左右的发射带源于浅施主能级到深受主能级间的施主-受主对辐射发光.【期刊名称】《发光学报》【年(卷),期】2014(035)005【总页数】4页(P585-588)【关键词】GaN纳米粒子;直接氮化法;光致发光【作者】沈龙海;富松;石广立【作者单位】沈阳理工大学理学院,辽宁沈阳110159;沈阳理工大学理学院,辽宁沈阳110159;沈阳理工大学理学院,辽宁沈阳110159【正文语种】中文【中图分类】O471.4;O482.311 引言GaN是一种优异的直接带隙半导体材料,室温下禁带宽度为3.4 eV,具有优良的光电性能、热稳定性及化学稳定性,是制作高亮度蓝绿发光二极管(LED)、激光二极管(LD)以及大功率、高温、高速和恶劣环境条件下工作的光电子器件的理想材料[1-2]。
最近有报道发现GaN基纳米材料具有吸收可见光使水解离产生氢的性能,这使得GaN纳米材料的研究获得了很多的关注[3-4]。
半导体纳米粒子由于小尺寸效应,往往会呈现不同于体材料的发光特性[5]。
但要实现高效可靠的光发射,尤其是可在柔性衬底上制作器件并可供日常使用的光发射材料仍然是个巨大的挑战[6]。
这使得GaN纳米材料的发光特性、能带结构及深能级行为的研究对其在未来纳米器件上的应用具有十分重要的现实意义。
目前,合成GaN纳米粒子方法主要有氨热法[7]、金属有机化合物化学气相沉积(MOCVD)法[8]、高温热解法[9-10]、胶体化学法[11]等。
MOCVD法生长硅基六方相GaN薄膜及其性质研究
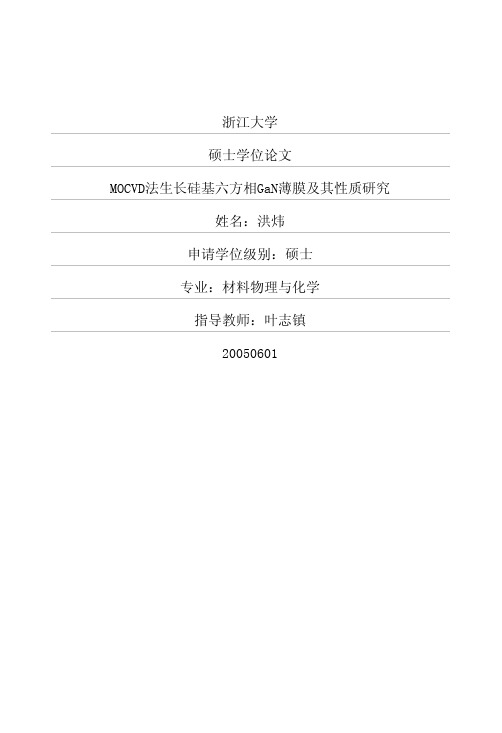
浙江大学硕士学位论文MOCVD法生长硅基六方相GaN薄膜及其性质研究姓名:洪炜申请学位级别:硕士专业:材料物理与化学指导教师:叶志镇20050601B^B^BAlatticeparameterlAI图2一lⅡI族氮化物的带隙宽度和晶格常数图2-2GaN晶体两种结构的原子排列示意图NGa(zzl)相对于传统的111.V族化合物半导体和硅,IⅢ英氮化物在全色显示、激光打印、高密度信息存储、水底通讯、自动控制引擎等领域应用前景十分广泛。
另由于IIl族氮化物带隙宽度大和化学键强,特别适合制作紫光、蓝光和绿光发光二极管以及高温、大功率器件。
GaN是一种极性晶体,由于Ga元素和N元素之间有较大的电负性差异(Ga=I.13,N=3.00)导致在GaN材料中存在很强的化学键,这是GaN具有许多独特物理性质的根源。
表2.1详细列出了GaN的一些物理性质。
的GaN样品。
系统的衬底转动部分采用磁流体密封技术,最高转速可达i500rpm。
生长室的本底真空度为5.0x10"SPa。
材料生长过程中各工艺参数均可通过计算机实现自动化控制。
此系统已申请国家发明专利(申请号03116768.3),并已通过浙江省科技厅组织的科技成果鉴定(浙科鉴字[2003]第199号).该设备由气体输送系统、系统腔体(包括生长室和进样室)、计算机控制系统、尾气排放系统组成。
图3一l所示为MOCVD系统的气路原理图。
高纯H2或N2作为载气可由水平与垂直两个方向进入生长室。
水平方向为主气流,输送参与反应的源。
垂直方向为副气流,输送N2与H2混合气体。
副气流的作用是克服由于衬底的高温而在其上方形成的气体对流,把主反应气体压向衬底,促进表面薄膜的二维生长。
各种气体或源的流量可由质量流量计精确控制。
图3-IMOCVD系统气路图图3-2为MOCVD系统腔体,它分为生长室A与进样室B两个部分。
生长li{f,衬底放入迸样室B后抽真空,而后打开中间的闸板阀l,再由机械手1将样品送入生长室A,然后再用机械手2将样品置于加热器上方托盘4上,关闭闸板阀l,生长室A继续抽真窑。
Ag作催化剂制备的GaN的形貌及其性能_朱琳
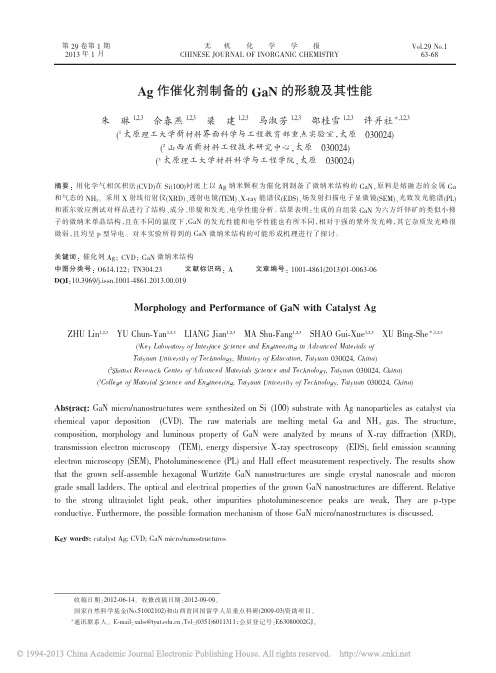
收稿日期:2012-06-14。
收修改稿日期:2012-09-09。
国家自然科学基金(No.51002102)和山西省回国留学人员重点科研(2009-03)资助项目。
*通讯联系人。
E -mail :xubs@ ,Tel :(0351)6011311;会员登记号:E63080002GJ 。
Ag 作催化剂制备的GaN 的形貌及其性能朱琳1,2,3余春燕1,2,3梁建1,2,3马淑芳1,2,3邵桂雪1,2,3许并社*,1,2,3(1太原理工大学新材料界面科学与工程教育部重点实验室,太原030024)(2山西省新材料工程技术研究中心,太原030024)(3太原理工大学材料科学与工程学院,太原030024)摘要:用化学气相沉积法(CVD)在Si(100)衬底上以Ag 纳米颗粒为催化剂制备了微纳米结构的GaN ,原料是熔融态的金属Ga 和气态的NH 3。
采用X 射线衍射仪(XRD)、透射电镜(TEM)、X -ray 能谱仪(EDS)、场发射扫描电子显微镜(SEM)、光致发光能谱(PL)和霍尔效应测试对样品进行了结构、成分、形貌和发光、电学性能分析。
结果表明:生成的自组装GaN 为六方纤锌矿的类似小梯子的微纳米单晶结构,且在不同的温度下,GaN 的发光性能和电学性能也有所不同,相对于强的紫外发光峰,其它杂质发光峰很微弱,且均呈p 型导电。
对本实验所得到的GaN 微纳米结构的可能形成机理进行了探讨。
关键词:催化剂Ag ;CVD ;GaN 微纳米结构中图分类号:O614.122;TN304.23文献标识码:A文章编号:1001-4861(2013)01-0063-06DOI :10.3969/j.issn.1001-4861.2013.00.019Morphology and Performance of GaN with Catalyst AgZHU Lin 1,2,3YU Chun -Yan 1,2,3LIANG Jian 1,2,3MA Shu -Fang 1,2,3SHAO Gui -Xue 1,2,3XU Bing -She *,1,2,3(1Key Laboratory of Interface Science and Engineering in Advanced Materials of Taiyuan University of Technology,Ministry of Education,Taiyuan 030024,China )(2Shanxi Research Center of Advanced Materials Science and Technology,Taiyuan 030024,China )(3College of Material Science and Engineering,Taiyuan University of Technology,Taiyuan 030024,China )Abstract:GaN micro/nanostructures were synthesized on Si (100)substrate with Ag nanoparticles as catalyst via chemical vapor deposition (CVD).The raw materials are melting metal Ga and NH 3gas.The structure,composition,morphology and luminous property of GaN were analyzed by means of X -ray diffraction (XRD),transmission electron microscopy (TEM),energy dispersive X -ray spectroscopy (EDS),field emission scanning electron microscopy (SEM),Photoluminescence (PL)and Hall effect measurement respectively.The results show that the grown self -assemble hexagonal Wurtzite GaN nanostructures are single crystal nanoscale and micron grade small ladders.The optical and electrical properties of the grown GaN nanostructures are different.Relative to the strong ultraviolet light peak,other impurities photoluminescence peaks are weak,They are p -type conductive.Furthermore,the possible formation mechanism of those GaN micro/nanostructures is discussed.Key words:catalyst Ag;CVD;GaN micro/nanostructures第29卷第1期2013年1月Vol .29No .163-68无机化学学报CHINESE JOURNAL OF INORGANIC CHEMISTRY第29卷无机化学学报0引言GaN是一种直接宽带隙半导体材料,它在蓝光、紫外光光电子器件以及高温、高频电子器件等方面具有广泛的应用前景[1-2]。
氮化铝的透光率

氮化铝的透光率1. 引言氮化铝(AlN)是一种具有广泛应用潜力的宽禁带半导体材料。
与其他材料相比,氮化铝具有优异的热导率、机械强度和化学稳定性。
此外,氮化铝的透光率也是其独特之处,对于光电器件的应用具有重要意义。
2. 氮化铝的透光率测量方法在研究氮化铝的透光率时,一种常用的方法是透射光谱法。
透射光谱法是通过测量材料对不同波长或频率的光的透射率来确定其透光率。
具体步骤如下:1.准备样品:将氮化铝样品切割成合适尺寸,并进行表面处理,以消除表面缺陷对透光率测试的影响。
2.设置实验装置:将氮化铝样品放置在透明基底上,使其成为一个光学胶片。
通过光源照射样品,并使用光探测器测量透射光信号。
3.测量透射光谱:通过改变光源的波长或频率,测量样品对不同波长或频率的光的透射率。
可以使用透射光谱仪或光谱分析仪进行测量。
4.数据处理:将测量得到的透射光谱数据进行处理,得到氮化铝在不同波长或频率下的透光率曲线。
3. 氮化铝透光率的影响因素氮化铝的透光率受多种因素影响,下面列举了几个主要因素:3.1 材料纯度氮化铝的纯度对其透光率有重要影响。
较高纯度的氮化铝样品通常具有更高的透光率。
杂质和缺陷会散射光线,导致透光率下降。
3.2 晶体结构氮化铝具有多种晶体结构,包括六方晶体和立方晶体。
不同晶体结构的氮化铝具有不同的透光率特性。
晶体结构的选择和优化可以改善氮化铝的透光率。
3.3 厚度氮化铝样品的厚度对其透光率有一定影响。
通常情况下,较薄的氮化铝样品具有较高的透光率。
随着厚度增加,光的散射和吸收会增加,透光率逐渐下降。
3.4 表面处理氮化铝样品的表面处理对其透光率也有一定影响。
不平整的表面会导致光的散射和吸收增加,降低透光率。
通过表面处理可以减少表面缺陷,提高透光率。
4. 氮化铝透光率的应用氮化铝具有优异的透光率,在光电器件的应用中具有广泛的应用前景。
以下是一些典型应用领域:4.1 光电子学氮化铝可以用于制造光电子器件,如激光二极管和光电传感器。
在蓝宝石衬底上外延生长GaN薄膜的MOCVD工艺研究
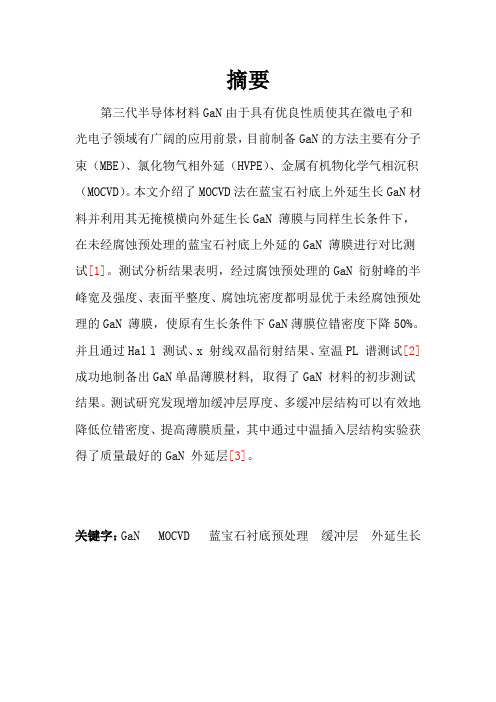
摘要第三代半导体材料GaN由于具有优良性质使其在微电子和光电子领域有广阔的应用前景,目前制备GaN的方法主要有分子束(MBE)、氯化物气相外延(HVPE)、金属有机物化学气相沉积(MOCVD)。
本文介绍了MOCVD法在蓝宝石衬底上外延生长GaN材料并利用其无掩模横向外延生长GaN 薄膜与同样生长条件下,在未经腐蚀预处理的蓝宝石衬底上外延的GaN 薄膜进行对比测试[1]。
测试分析结果表明,经过腐蚀预处理的GaN 衍射峰的半峰宽及强度、表面平整度、腐蚀坑密度都明显优于未经腐蚀预处理的GaN 薄膜,使原有生长条件下GaN薄膜位错密度下降50%。
并且通过Hal l 测试、x 射线双晶衍射结果、室温PL 谱测试[2]成功地制备出GaN单晶薄膜材料, 取得了GaN 材料的初步测试结果。
测试研究发现增加缓冲层厚度、多缓冲层结构可以有效地降低位错密度、提高薄膜质量,其中通过中温插入层结构实验获得了质量最好的GaN 外延层[3]。
关键字:GaN MOCVD 蓝宝石衬底预处理缓冲层外延生长STUDY OF EPITAXIAL LATERAL OVERGROWTH OF GALLIUM NITRIDE ON SAPPHIRE BYMOCVDByHaiqing JiangSupervisor: Prof.Xianying DaiABSTRACTGallium-nitride-semiconductor offers good potential value for application in a wide range of optical display, optical recording and illumination due to its excellent quality. At present, molecular beam epitaxity (MBE), Chloride vapor phase epitaxy (HVPE) and metal organic chemical vapor deposition (MOCVD) are used to prepare GaN.This text introduces overgrowth of Gallium-nitride on sapphire by MOCVD and compares the result with that on non-corrode sapphire. The results proved that thinner full-width at half- maximum(FWHM),higher intensity value of X-ray diffraction,smoother surface and lower density value of the etching pit were received using patterned substrate, which made sure that under the same growth process the density of the dislocations decreased 50%.After that, it also uses Hall Test, X-ray macle diffraction Test, and PL Spectrum Test under room temperature to check the GaN thin-film material. The results showed that multi-buffer-layer structure could decrease the density of the dislocations and improve the quality of the crystal structure. The GaN epilayer with Intermediate-Temperature insert layer had the best results of all the samples.KEY WORDS: GaN MOCVD surface pretreatment on sapphire substrate cushion epitaxial growth第一章绪论1.1GaN 材料的基本特性1.2现有的GaN 基化合物的制备技术1.3GaN 现有制备技术对比第二章 MOCVD 中影响成膜因素第三章蓝宝石衬底表面预处理3.1蓝宝石衬底与处理的原因3.2实验探究与结果分析第四章研究缓冲层结构及其改进4.1传统缓冲层及其局限4.2实验探究及其结果分析第五章GaN 薄膜的生长研究5.1GaN材料的生长5.2生长的GaN 材料的测试结果第六章结论致谢参考文献第一章绪论1.1GaN 材料的基本特性GaN 首先由Johnson 等人合成,合成反应发生在加热的Ga 和NH3 之间,600~900℃的温度范围,可生成白色、灰色或棕色粉末(是含有O 或未反应的Ga 所致)[4]。
LPE生长GaN的不同极性面的光学性质
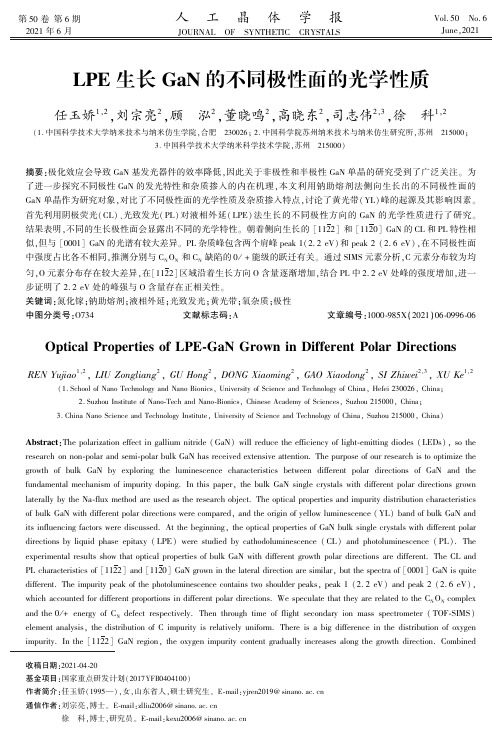
Abstract:The polarization effect in gallium nitride ( GaN) will reduce the efficiency of light-emitting diodes ( LEDs) , so the research on non-polar and semi-polar bulk GaN has received extensive attention. The purpose of our research is to optimize the growth of bulk GaN by exploring the luminescence characteristics between different polar directions of GaN and the fundamental mechanism of impurity doping. In this paper, the bulk GaN single crystals with different polar directions grown laterally by the Na-flux method are used as the research object. The optical properties and impurity distribution characteristics of bulk GaN with different polar directions were compared, and the origin of yellow luminescence ( YL) band of bulk GaN and its influencing factors were discussed. At the beginning, the optical properties of GaN bulk single crystals with different polar directions by liquid phase epitaxy ( LPE) were studied by cathodoluminescence ( CL) and photoluminescence ( PL) . The experimental results show that optical properties of bulk GaN with different growth polar directions are different. The CL and PL characteristics of [1122] and [1120] GaN grown in the lateral direction are similar, but the spectra of [0001] GaN is quite different. The impurity peak of the photoluminescence contains two shoulder peaks, peak 1 (2. 2 eV) and peak 2 (2. 6 eV), which accounted for different proportions in different polar directions. We speculate that they are related to the CN ON complex and the 0 / + energy of CN defect respectively. Then through time of flight secondary ion mass spectrometer ( TOF-SIMS) element analysis, the distribution of C impurity is relatively uniform. There is a big difference in the distribution of oxygen impurity. In the [1122] GaN region, the oxygen impurity content gradually increases along the growth direction. Combined
GaN基半导体材料的非线性光学性质研究的开题报告
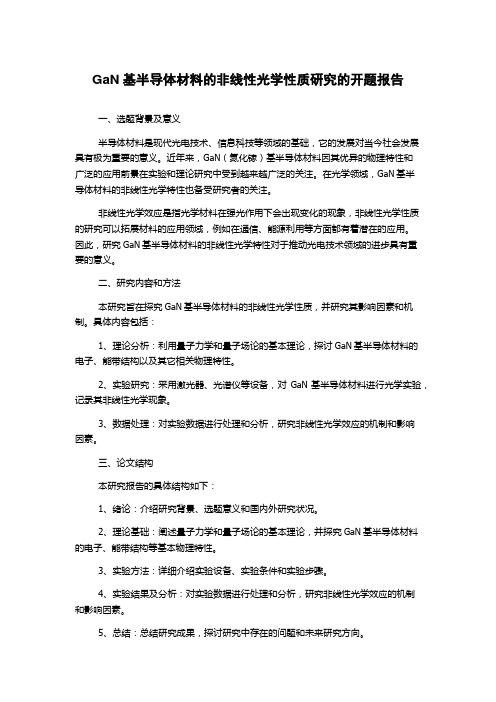
GaN基半导体材料的非线性光学性质研究的开题报告一、选题背景及意义半导体材料是现代光电技术、信息科技等领域的基础,它的发展对当今社会发展具有极为重要的意义。
近年来,GaN(氮化镓)基半导体材料因其优异的物理特性和广泛的应用前景在实验和理论研究中受到越来越广泛的关注。
在光学领域,GaN基半导体材料的非线性光学特性也备受研究者的关注。
非线性光学效应是指光学材料在强光作用下会出现变化的现象,非线性光学性质的研究可以拓展材料的应用领域,例如在通信、能源利用等方面都有着潜在的应用。
因此,研究GaN基半导体材料的非线性光学特性对于推动光电技术领域的进步具有重要的意义。
二、研究内容和方法本研究旨在探究GaN基半导体材料的非线性光学性质,并研究其影响因素和机制。
具体内容包括:1、理论分析:利用量子力学和量子场论的基本理论,探讨GaN基半导体材料的电子、能带结构以及其它相关物理特性。
2、实验研究:采用激光器、光谱仪等设备,对GaN基半导体材料进行光学实验,记录其非线性光学现象。
3、数据处理:对实验数据进行处理和分析,研究非线性光学效应的机制和影响因素。
三、论文结构本研究报告的具体结构如下:1、绪论:介绍研究背景、选题意义和国内外研究状况。
2、理论基础:阐述量子力学和量子场论的基本理论,并探究GaN基半导体材料的电子、能带结构等基本物理特性。
3、实验方法:详细介绍实验设备、实验条件和实验步骤。
4、实验结果及分析:对实验数据进行处理和分析,研究非线性光学效应的机制和影响因素。
5、总结:总结研究成果,探讨研究中存在的问题和未来研究方向。
四、预期成果本研究预期可以:1、深入研究GaN基半导体材料的非线性光学性质,为其实际应用提供理论依据。
2、采用激光器等先进设备进行实验研究,得到具有实际意义的数据和结果。
3、发表高质量的学术论文,为相关领域的研究和实际应用提供参考。
五、可能面临的问题和解决方案在研究过程中,可能会面临以下问题:1、实验条件相对复杂,需要较高的实验技能和仪器使用能力。
- 1、下载文档前请自行甄别文档内容的完整性,平台不提供额外的编辑、内容补充、找答案等附加服务。
- 2、"仅部分预览"的文档,不可在线预览部分如存在完整性等问题,可反馈申请退款(可完整预览的文档不适用该条件!)。
- 3、如文档侵犯您的权益,请联系客服反馈,我们会尽快为您处理(人工客服工作时间:9:00-18:30)。
Optical properties of GaN grown on Si…111…by gas source molecular beam epitaxy with ammoniaA.S.Zubrilov,a)S.A.Nikishin,b)G.D.Kipshidze,V.V.Kuryatkov,and H.TemkinDepartment of Electrical Engineering,Texas Tech University,Lubbock,Texas79409T.I.Prokofyeva and M.HoltzDepartment of Physics,Texas Tech University,Lubbock,Texas79401͑Received13July2001;accepted for publication29October2001͒We report a study of the optical properties of GaN grown on Si͑111͒by gas source molecular beamepitaxy with ammonia.Temperature dependence of edge luminescence was studied in the range of77–495K for samples with low background carrier concentrations,as determined by capacitancevoltage profiling and Raman spectroscopy,and the results werefitted using Passler’s and Varshni’smodels.We also demonstrate strong correlation between electron concentration in GaN and relativeRaman intensity of A1͑longitudinal optical͒and E22modes.The binding energy of free excitons isestimated to be29Ϯ2meV.The contributions of different mechanisms to free exciton linebroadening are discussed.©2002American Institute of Physics.͓DOI:10.1063/1.1430535͔INTRODUCTIONSilicon is a very attractive substrate for the growth of gallium nitride due to advantages such as low cost,well-developed postgrowth processes,high thermal conductivity, and large diameter of available wafers.1Nitride-based device structures grown on silicon by molecular beam epitaxy ͑MBE͒have been recently demonstrated.2–8Using gas source MBE͑GSMBE͒with ammonia,nitride layers can be grown at high temperatures,9,10required to achieve device-quality optical and electrical properties.11GSMBE can be used to grow high quality AlN12,13and GaN14–16on Si͑111͒.High growth temperature for AlN, combined with carefully controlled formation of the Si–N–Al interface layer16at the onset of epitaxy,result in very rapid transition to two-dimensional growth mode of AlN and the subsequent GaN.Additional short period blocking super-lattices͑SLs͒of AlGaN/GaN and GaN spacers reduce dislo-cation propagation to the upper GaN layer.17They also sta-bilize residual tensile stress level and the resulting layers are free of cracks.14All GaN layers studied here were grown at a temperature of1050Ϯ20K and growth rates of0.4–1.1m/h.Formation of a(2ϫ2)surface structure,characteristic of two-dimensional growth,could be seen after deposition of 20–100nm of GaN.The total thickness of the GaN samples varied from0.4to2.5m.Details of the growth can be found elsewhere.12,14,16,17While the structural perfection of nitride layers grown by GSMBE on Si͑111͒is by now well established,17,18the opti-cal properties,such as temperature dependence of the band gap and the contribution of different mechanisms to free ex-citon line broadening are not well known.In this article we describe optical studies of high quality GaN layers grown on Si͑111͒by GSMBE with ammonia.For this investigation we select samples with low background carrier concentration.Since the background electron concen-tration varies from low1016to high1018cmϪ3,depending on growth conditions,careful sample selection is needed to distinguish intrinsic optical properties.When nitride layers are grown on Si substrates,which are conducting,determi-nation of their carrier levels is not simple.19We use a com-bination of capacitance–voltage profiling,with mercury and gold Schottky barriers,and Raman spectroscopy for sample selection.Optical properties of samples with the background electron concentration ofϳ(1–5)ϫ1016cmϪ3were inves-tigated by photoluminescence͑PL͒excited by a2kW pulse ͑10ns,100Hz͒nitrogen laser operating at a wavelength of 337.1nm.Sample temperature was varied from77to495K.Raman measurements were made at room temperature using488.0nm laser excitation.Spectra were collected in a direct backscattering z(y,y)z¯polarization configuration us-ing a Raman microprobe.20Wefind that Raman measure-ments can be used to estimate free carrier concentration.This technique has the advantage of being rapid and contactless. Figure1shows a series of Raman spectra taken from GaN/Si samples having different free-carrier concentrations n due to the growth under stoichiometric and nonstoichiometric conditions.21Spectrum1is that of a sample grown at sto-ichiometric conditions and growth rate of0.57m/h.This growth rate was found to result in the lowest background level.The narrow E22phonon,at565cmϪ1,dominated all spectra.A weaker band was observed near733cmϪ1.At low n this peak is related to the A1longitudinal optical͑LO͒phonon.As the free-carrier concentration increases͑spec-trum2and3͒,this band shows reduced intensity,and exhib-its a broader,asymmetric shoulder at higher energy.LO phonons in polar semiconductors interact with free-carrier plasmas to produce mixed LO phonon–plasmon bands,usually denoted by LϪand Lϩ.22The Lϩappears ata͒Permanent address:Ioffe Institute,St.Petersburg,Russia,194021;elec-tronic mail:asz@pop.ioffe.rssi.rub͒Author to whom correspondence should be addressed;electronic mail:sergey.nikishin@JOURNAL OF APPLIED PHYSICS VOLUME91,NUMBER31FEBRUARY200212090021-8979/2002/91(3)/1209/4/$19.00©2002American Institute of Physicsthe A 1(LO)phonon frequency at low carrier concentrations,and blueshifts with increasing n .We used the well-known model applied to GaN in Ref.23to determine the carrier concentration in our GaN samples based on the L ϩenergy and line shape.The filled circles of Fig.2compare the values of n determined from analyzing the L ϩband with those ob-tained from Capacitance-V oltage (C –V )measurements.The agreement is excellent.However,the analysis fails at con-centrations below 4ϫ1016cm Ϫ3because the L ϩbecomes indistinguishable from the A 1(LO)phonon.The presence of the A 1(LO)phonon band,which should not appear due to the interaction with free-carrier plasmas,can be attributed to the existence of a near-surface depletion region.24Since the scattering intensity of the residual A 1(LO)is proportional to the width of the depletion region,it decreases with increasing n .Assuming that the E 22phonon intensity is directly proportional to the total epilayer thick-ness d of the transparent GaN layer,we can use this intensity as an internal standard provided we normalize it by d .When normalized this way,the relative intensity I rel ϭI (A 1)/(I (E 2)/d )should be proportional to the width of the deple-tion region.The open circles of Fig.2show the dependence of the relative intensity on n determined by C –V .The poly-nomial fitting of this dependence yields:log(n )ϭ16.0Ϫ2.6a Ϫ0.735a 2,where a ϭlog(I rel ).The dependence is not simple at high carrier concentrations,because the Fermi level can be influenced by surface states present,for example,due to oxi-dation.From our analysis,relative intensity can be used as a rapid means for estimating free-carrier concentrations in ep-itaxial ͑less than 8m thick ͒n -type GaN without the depo-sition of contacts.The calibration in Fig.2is valid for 488nm excitation and z (y ,y )z ¯polarization configuration.The calibration changes with either a different laser wavelength or polarization condition.The Raman measurements were used to identify suitable samples for PL study.Narrow line-width of the E 22phonon demonstrated high sample quality in all cases,and the E 22energy allowed us to determine the stress.The stress was found to be р0.3GPa and independent of epilayer thickness when an AlN buffer layer,followed by a series of short-period SLs separated by GaN spacers,14was used.The room temperature PL spectrum of the best GaN samples shows a free exciton ͑FE ͒peak at 3.408eV with full width half maximum ͑FWHM ͒of less than 40meV and weak ‘‘yellow’’luminescence at ϳ2.2eV .We did not find strong dependence of either the exciton peak or ‘‘yellow’’luminescence intensity on the number of blocking SL and GaN spacer layers.PL spectra taken at different temperatures in the range from 77to 495K are presented in Fig.3.At elevated temperatures (T Ͼ100K)the PL spectra are domi-nated by the FE recombination.25,26At temperatures below 100K bound exciton-related optical transitions contribute to the edge peak spectral contour.25Integrated PL emission intensity I of the FE band versus temperature is presented in Fig.4͑a ͒.The intensity of FE transition decreases with increased temperature due to ther-mal activation,and can be described byI ͑T ͒ϭI 0/͓1ϩexp ͑ϪE a /kT ͔͒,͑1͒where we assume the same activation energy for A (n ϭ1)and B (n ϭ1)excitons.27The fitted value of the activation energy E a is 29Ϯ2meV,in agreement with published data for A -exciton binding energy.28,29Some deviation of experi-mental I (T )dependence from the exponential law athighFIG.1.Room temperature Raman spectra of GaN with different back-ground electron concentrations.The spectra are normalized to the same E 22intensity.͑1͒GaN grown under stoichiometric conditions and optimal growth rate of ϳ0.57m/h;n ϭ1.3ϫ1016cm Ϫ3;͑2͒GaN grown under stoichiometric conditions and high growth rate of ϳ0.9m/h;n ϭ1.5ϫ1017cm Ϫ3;and ͑3͒GaN grown under Ga-rich condition and growth rate of ϳ0.5m/h;n ϭ1.0ϫ1018cm Ϫ3.FIG.2.Correlation between electron concentration,obtained from C –V measurements,and relative Raman intensity ͑open circles ͒,and from analy-sis of the phonon–plasmon coupled mode ͑filled circles ͒.I REL is the relative intensity of the A 1͑LO ͒phonon band to that of the E 2phonon normalized to the layer thickness.With this calibration curve we estimate carrier con-centration using a polynomial fit log(n )ϭ16.0–2.6a Ϫ0.735a 2.FIG.3.PL spectra of GaN/Si ͑111͒taken as a function of temperature.temperatures (T Ͼ450K)can be explained by nonradiative recombination processes.The value obtained for E a can be used to estimate the band gap of GaN as the sum of the FE energy plus the value of E a (E g ϭE FE ϩE a ).The main mechanisms of the temperature induced gap shrinkage are:͑i ͒a change of lattice parameters ͑this also occurs when pres-sure is applied to a crystal ͒and ͑ii ͒the electron–phonon interaction.According to Ref.30the first mechanism con-tributes about 14%of the total E g shrinkage in bulk GaN.Thus,the second mechanism is dominant.The temperature dependence of the band gap is presented in Fig.4͑b ͒.The solid line ͑1͒represents the best fit to Passler’s model:31E g (T )ϭE g (0)Ϫ(␣⌰/2)͕͓1ϩ(2T /⌰)p ͔1/p Ϫ1͖,where E g (0)ϭ3.495eV is the band gap at T ϭ0K,␣ϭ0.53meV/K is the gap shrinkage coefficient,⌰ϳ400K is the effective phonon temperature,and p ϭ2.6.Here p ϭϩ1is the power coefficient from the power law electron–phonon spectral function f ()ϭ(␣/k )(/0)and ϵh is phonon energy.At temperatures higher than ⌰(T ӷ⌰)the electron–phonon interaction mechanism is approximately linear.At low temperatures (T Ӷ⌰)this mechanism is very weak.Ac-cording to Ref.31,the effective phonon temperatures of group IV ,III–V ,and II–VI materials are nearly proportional to the respective Debye temperatures (⌰D ):⌰ϳ(2/3)⌰D .The fitted value of ⌰ϭ400K is in good agreement with this relation and the known ⌰D ϳ600K.32Varshni’s 33approxi-mation ͓Fig.4͑b ͒,curve 2͔for the band gap energy shrinkage (E g (T )ϭE g (0)Ϫ␥T 2/(T ϩ))gives the following param-eters:␥ϭ0.77meV/K,ϭ600K.Here ␥represents the T →ϱlimit of ϪdE g (T )/dT ,and is comparable to the De-bye temperature ⌰D .The fitted parameters are in good agreement with those found previously for GaN layers grown on SiC and sapphire substrates ͑see Table I ͒.The temperature dependence of the band gap energy of a GaN layer grown by metalorganic chemical vapor deposition on 6H–SiC substrate 34and of a stress-free bulk GaN crystal grown by HVPE 35are also presented in Fig.4͑b ͒as lines 3and 4,respectively.GaN grown on Si lies closer to the stress-free GaN than does the GaN grown on SiC.We attribute this to lower stress.Stress of ϳ0.5–1GPa arises in GaN/SiC primarily due to differences in thermal expansion coeffi-cients of GaN and SiC and high growth temperature.We estimate the tensile stresses in our GaN/Si samples to be in the range 0.08–0.3GPa,which is in good agreement with our Raman measurements.14The temperature dependence of the FWHM of the FE recombination peak is presented in Fig.4͑c ͒.At low tem-perature the dependence is linear.At higher temperatures ͑above 200K ͒a deviation from the linear dependence is observed.The FE linewidth can be described by 36⌫1/2ϭ⌫I ϩ⌫ac ϩ⌫opt ,͑2͒where ⌫I is the inhomogeneous linewidth due to defects and strain,⌫ac is the contribution from acoustic phonon scatter-ing of the excitons (⌫ac ϭT ),and ⌫opt is due to exciton scattering by LO phonons.The effect of exciton scattering can be described by ⌫opt ϭ/͓exp(E LO /kT )Ϫ1͔.37The best fit of Eq.͑2͒to our data is shown in the inset of Fig.4,and the parameters used are listed in Table II.The results of our analysis are in good agreement with those reported in Ref.36.However,their linewidth ⌫1is smaller,possibly due to lower defect concentration or lower inhomogeneous stress.In conclusion,the GaN/Si material grown by GSMBE with ammonia 14exhibits low stress.The temperature depen-dence of the band gap is close to that of stress free bulk GaN crystals and well described by Passler’s and Varshni’s mod-els.We studied the contributions of different mechanisms of the FE line broadening.At low temperatures of ϳ100–300KFIG.4.Temperature dependences of intensity of exiton peak,band gap energy,and FWHM of exiton peak:͑a ͒the temperature dependence of the integrated PL intensity of the FE peak:͑squares ͒experiment;͑curves ͒cal-culations for different activation energies in Eq.͑1͒͑from 19to 37meV ͒.The best fit corresponds to E a ϭ29Ϯ2meV;͑b ͒the experimental tempera-ture dependence of the band gap energy ͑shown by circles ͒.Line ͑1͒Passler’s model fit for parameters listed in the text;͑2͒Varshni’s model fit;͑3͒GaN layer ͑under tension ͒grown by MOCVD on SiC ͑data recalculated from Ref.34͒;͑4͒Strain free bulk GaN grown by HVPE ͑Ref.35͒.͑c ͒The temperature dependence of FWHM of the exciton-related PL peak,͑squares ͒experiment,͑curve ͒best fit of Eq.͑2͒.TABLE I.Parameters used to fit Varshni’s equation:E g (T )ϭE g (0)Ϫ␥T 2/(T ϩ).Sample type ␥͑meV/K ͒͑K ͒Ref.GaN/Si ͑111͒0.77600This workGaN/sapphire 0.7260038GaN/sapphire 0.93977230Bulk GaN crystal 0.7460034Bulk GaN crystal1.0874530TABLE II.Parameters used to fit Eq.͑2͒.⌫I ͑meV ͒͑meV/K ͒͑meV ͒Reference 11.50.035100This work20.01821036the dominant line broadening mechanism is the inhomoge-neous line broadening due to defects and inhomogeneous stress,while at high temperature above400K the dominant line broadening mechanism is the effect of exciton scattering with LO phonons.We show strong correlation between elec-tron concentration in GaN,obtained from C–V measure-ments and relative Raman intensity of A1(LO)and E22modes of GaN.ACKNOWLEDGMENTSThe authors would like to acknowledge discussions with Dr.Vladimir Dmitriev.Work at TTU is supported by DARPA,NSF͑Grant No.ECS-0070240͒,CRDF͑Grant No. RE1-2217͒,NATO Science for Peace Program͑Grant No. SfP-974505͒,and J.F.Maddox Foundation.1E.S.Hellman,D.N.E.Buchanan,and C.H.Chen,MRS Internet J. Nitride Semicond.Res.3,43͑1998͒.2O.Aktas,W.Kim,Z.Fan,A.Botchkarev,A.Salvador,S.N.Mohammad, B.Sverdlov,and H.Morkoc¸,Electron.Lett.31,1389͑1995͒.3R.J.Molnar,R.Singh,and T.Moustakas,Appl.Phys.Lett.66,268͑1995͒.4N.Grandjean,J.Massies,M.Leroux,and P.Lorenzini,Appl.Phys.Lett. 72,82͑1998͒.5S.Guha and N.A.Bojarzuk,Appl.Phys.Lett.72,415͑1998͒.6I.P.Smorchkova et al.,Appl.Phys.Lett.76,718͑2000͒.7M.A.Sa´nchez-Garcı´a,F.B.Narajo,J.L.Pau,A.Jimenez,E.Calleja,and E.Mun˜oz,J.Appl.Phys.87,1569͑2000͒.8P.W.Deelman,R.N.Bicknell-Tassius,S.A.Nikishin,V.V.Kuryatkov, and H.Temkin,Appl.Phys.Lett.78,2172͑2001͒.9N.Grandjean,J.Massies,B.Damilano,S.Yu.Karpov,and R.A.Talalaev, Appl.Phys.Lett.74,1854͑1999͒.10S.Yu.Karpov,R.A.Talalaev,Yu.N.Makarov,N.Grandjean,J.Massies, and B.Damilano,Surf.Sci.450,191͑2000͒.11H.Tang and J.B.Webb,Appl.Phys.Lett.74,2373͑1999͒.12S.A.Nikishin et al.,Appl.Phys.Lett.75,484͑1999͒.13G.Kipshidze,H.P.Schenk,A.Fissel,U.Kaiser,J.Schulze,Wo.Richter, M.Weihnacht,R.Kunze,and J.Kra¨usslich,Semiconductors33,1241͑1999͒.14S.A.Nikishin et al.,Appl.Phys.Lett.75,2073͑1999͒.15F.Semond,P.Lorenzini,N.Grandjean,and J.Massies,Appl.Phys.Lett. 78,335͑2001͒.16S.A.Nikishin et al.,Mater.Res.Soc.Symp.Proc.595,W8.3.1-͑6͒͑2000͒.17S.A.Nikishin,N.N.Faleev,H.Temkin,and S.N.G.Chu,Proc.-Electrochem.Soc.99-17,238͑1999͒.18R.Rinaldi,S.Antonaci,M.Anni,M.Lomascolo,R.Cingolani,A.Botch-karev,and H.Morkoc,Phys.Status Solidi B216,701͑1999͒.19E.Calleja et al.,Phys.Rev.B58,1550͑1998͒.20M.Holtz,M.Seon,T.Prokofyeva,H.Temkin,R.Singh,F.P.Dabkowski, and T.D.Moustakas,Appl.Phys.Lett.75,1757͑1999͒.21S.Nikishin et al.,J.Vac.Sci.Technol.B19,1409͑2001͒.22A.Mooradian and G.B.Wright,Phys.Rev.Lett.16,999͑1966͒.23T.Kozawa,T.Kachi,H.Kano,Y.Taga,M.Hashmoto,N.Koide,and K. Manabe,J.Appl.Phys.75,1098͑1993͒.24A.Pinczuk and E.Burstein,Phys.Rev.Lett.21,1073͑1968͒.25B.Monemar,J.P.Bergman,I.A.Buyanova,W.Li,H.Amano,and I. Akasaki,MRS Internet J.Nitride Semicond.Res.1,2͑1996͒.26M.Smith,G.D.Chen,J.K.Lin,H.X.Jiang,M.Asif Khan,C.J.Sun,Q. Chen,and J.W.Yang,J.Appl.Phys.79,7001͑1996͒.27D.Bimberg,M.Sondergeld,and E.Grobe,Phys.Rev.B4,3451͑1971͒. 28D.Kovalev,B.Averboukh,D.V olm,B.K.Meyer,H.Amano,and I. Akasaki,Phys.Rev.B54,2518͑1996͒.29M.Leroux,N.Grandjean,B.Beaumont,G.Nataf,F.Semond,J.Massies, and P.Gibart,Phys.Status Solidi B216,605͑1999͒.30H.Teisseyere,P.Perlin,T.Susuki,I.Grzegory,S.Porovski,J.Jun,A. Pietraszko,and T.D.Moustakas,J.Appl.Phys.76,2429͑1994͒.31R.Passler,Phys.Status Solidi B200,155͑1997͒.32Properties of Advanced Semiconductor Materials GaN,AlN,InN,BN,SiC, SiGe.edited by M.E.Levinshtein,S.L.Rumyantsev,and M.S.Shur ͑Wiley,New York,2001͒,p.194.33Y.P.Varshni,Physica͑Amsterdam͒34,149͑1967͒.34A.S.Zubrilov,V.I.Nikolaev,D.V.Tsvetkov,V.A.Dmitriev,K.G. Irvine,J.A.Edmond,and C.H.Carter,Jr.,Appl.Phys.Lett.67,533͑1995͒.35A.S.Zubrilov,Yu.V.Melnik,A.E.Nikolaev,M.A.Jakobson,D.K. Nelson,and V.A.Dmitriev,Semiconductors33,1067͑1999͒.36B.Monemar,I.A.Buyanova,and J.P.Bergman.,Abstracts of ICSCIII-N’97,Stockholm,Sweden,August31–September5,1997,pp.676–677. 37S.Rudin,T.L.Reinicke,and B.Segall,Phys.Rev.B42,11218͑1990͒. 38M.Ilegems,R.Dingle,and R.A.Cogan,J.Appl.Phys.43,3797͑1972͒.。