土木外文翻译--高温下钢筋混凝土中钢筋的性能
土木工程外文文献翻译

土木工程外文文献翻译数学模型预测水运输混凝土结构中的渗透性eluozo,s.n全文粗骨料细砂的混凝土构件,大孔隙混凝土率确定孔隙率和混凝土结构孔隙比,渗透系数的影响确定率水运混凝土。
数学模型来预测渗透率对水率交通是数学发展,该模型是监测水运输的混凝土率结构。
渗透性建立大孔上构成的影响下一种关系,即由混凝土制成,应用混凝土浇筑的决定渗透性的沉积速率混凝土结构,渗透性建立是大孔的混合物之间的影响下通过水泥净浆,考虑到系统中的变量,数学模型的建立是为了监测水通过通过具体的速度,也确定渗透系数的率对混凝土结构。
关键字:混凝土结构、渗透性和数学模型一、简介混凝土结构的耐久性依赖于通过频密迁移率的熔化的成分。
这种搬迁就是通过磁导率的影响。
在排序该条件混凝土混合物就是通过中存有的基质中的微孔隙的已连续网络混凝土协调比。
其他影响就是通过存有于的界面的孔隙率骨料的级分体式结构。
本研究中,其特征测量的快速和精确度在煮混凝土的渗透性,这包含创建理论模型的叙述渗透性对混凝土结构的影响。
实验中采用的就是瞬时顺利完成渗透性设备监控措施细骨料细沙和水是这种材料例如混凝土称作孔隙率和孔隙率中的组件之间的微孔混凝土结构中,渗透系数的影响确认水的速率运输在混凝土加水物水分搬迁混凝土,设备容许快速和精确测量在混凝土加水水中搬迁。
混凝土就是一种类型的多孔材料做成,并且可以由于在物理上和化学受损其曝露在各种环境中从混凝土浇筑至其使用寿命。
在特别就是,一些外部有毒元素,例如硫酸根,氯离子,和二氧化碳,扩散在混凝土少于长期周期做为溶液或气体状态,并引致物理侵害,由于化学反应。
这些反应可以影响应用领域中钢筋破损具体内容的,这减少了耐热寿命,例如钢筋和力量。
因此,它是非常重要的是插入腐蚀抑制剂为在超过临界恶化元件的情况下钢棒腐蚀的钢筋的位置量[1]。
然而,这是非常困难的保证在使用该应用传统技术钢筋位置的耐腐蚀性腐蚀抑制剂仅在混凝土[2-3]的表面上。
土木毕业设计外文翻译--近表面埋置加固的钢筋混凝土梁抗弯性能实验研究
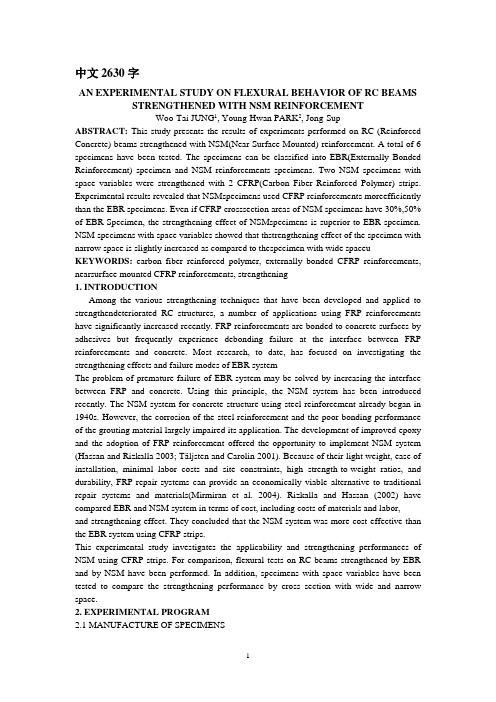
中文2630字AN EXPERIMENTAL STUDY ON FLEXURAL BEHAVIOR OF RC BEAMS STRENGTHENED WITH NSM REINFORCEMENTWoo-Tai JUNG1, Young-Hwan PARK2, Jong-SupABSTRACT: This study presents the results of experiments performed on RC (Reinforced Concrete) beams strengthened with NSM(Near Surface Mounted) reinforcement. A total of 6 specimens have been tested. The specimens can be classified into EBR(Externally Bonded Reinforcement) specimen and NSM reinforcements specimens. Two NSM specimens with space variables were strengthened with 2 CFRP(Carbon Fiber Reinforced Polymer) strips. Experimental results revealed that NSMspecimens used CFRP reinforcements moreefficiently than the EBR specimens. Even if CFRP crosssection areas of NSM specimens have 30%,50% of EBR Specimen, the strengthening effect of NSMspecimens is superior to EBR specimen. NSM specimens with space variables showed that thstrengthening effect of the specimen with narrow space is slightly increased as compared to thespecimen with wide spaceu KEYWORDS: carbon fiber reinforced polymer, externally bonded CFRP reinforcements, nearsurface mounted CFRP reinforcements, strengthening1. INTRODUCTIONAmong the various strengthening techniques that have been developed and applied to strengthendeteriorated RC structures, a number of applications using FRP reinforcements have significantly increased recently. FRP reinforcements are bonded to concrete surfaces by adhesives but frequently experience debonding failure at the interface between FRP reinforcements and concrete. Most research, to date, has focused on investigating the strengthening effects and failure modes of EBR systemThe problem of premature failure of EBR system may be solved by increasing the interface between FRP and concrete. Using this principle, the NSM system has been introduced recently. The NSM system for concrete structure using steel reinforcement already began in 1940s. However, the corrosion of the steel reinforcement and the poor bonding performance of the grouting material largely impaired its application. The development of improved epoxy and the adoption of FRP reinforcement offered the opportunity to implement NSM system (Hassan and Rizkalla 2003; Täljsten and Carolin 2001). Because of their light weight, ease of installation, minimal labor costs and site constraints, high strength-to-weight ratios, and durability, FRP repair systems can provide an economically viable alternative to traditional repair systems and materials(Mirmiran et al. 2004). Rizkalla and Hassan (2002) have compared EBR and NSM system in terms of cost, including costs of materials and labor,and strengthening effect. They concluded that the NSM system was more cost-effective than the EBR system using CFRP strips.This experimental study investigates the applicability and strengthening performances of NSM using CFRP strips. For comparison, flexural tests on RC beams strengthened by EBR and by NSM have been performed. In addition, specimens with space variables have been tested to compare the strengthening performance by cross section with wide and narrow space.2. EXPERIMENTAL PROGRAM2.1 MANUFACTURE OF SPECIMENSA total of 6 specimens of simply supported RC beams with span of 3m have been cast. The details andcross-section of the specimens are illustrated in Figure 1. A concrete with compressive strength of31.3 MPa at 28 days has been used. Steel reinforcements D10(φ9.53mm) of SD40 have been arrangedwith steel ratio of 0.0041 and a layer of three D13(φ12.7mm) has been arranged as compressionreinforcements. Shear reinforcements of D10 have been located every 10 cm in the shear zone to avoidshear failure. Table 1 summarizes the material properties used for the test beams.2.2 EXPERIMENTAL PARAMETERSTable 2 lists the experimental parameters. The control specimen, an unstrengthened specimen, has been cast to compare the strengthening performances of the various systems. CPL-50-BOND, EBR specimen, has been strengthened with CFRP strip. The remaining 4 specimens were strengthened with NSM CFRP strips. Among the specimens strengthened with NSM reinforcements, an embedding64 depth of NSM-PL-15 and NSM-PL-25 is 15mm and 25mm, respectively. A space between grooves of NSM-PL-25*2 and NSM-PL-2S is 60mm and 120mm, respectively. The strengthened length of all thespecimens has been fixed to 2,700 mm2.3 INSTALLATION OF THE FRP REINFORCEMENTSFigure 2 shows the details of cross-sections of the specimens. The strengthening process of EBR specimen (CPL-50-BOND) was proceeded by the surface treatment using a grinder, followed by the bonding of the CFRP strip. The strengthened beams were cured at ambient temperature for 7 days for the curing of epoxy adhesive. The process for NSM strengthening progressed by cutting the grooves at the bottom of the beams using a grinder, cleaning the debris, and embedding the CFRP strip after application of the adhesive. The strengthenedbeams were cured for 3 days so that the epoxy adhesive achieves its design strength.2.4 LOADING AND MEASUREMENT METHODSAll specimens were subjected to 4-point bending tests to failure by means of UTM (Universal Testing Machine) with capacity of 980 kN. The loading was applied under displacement control at a speed of 0.02 mm/sec until the first 15 mm and 0.05 mm/sec from15 mm until failure. The measurement of alltest data was recorded by a static data logger anda computer at intervals of 1 second. Electrical resistance strain gauges were fixed at mid-span and L/4 to measure the strain of steel reinforcements.Strain gauges to measure the strain of concrete were located at the top, 5 cm and 10 cm away from the top on one side at mid-span. Strain gauges were also placed on the FRP reinforcement located at the bottom of the mid-span and loaded points to measure the strain according to the loading process.3. EXPERIMENTAL RESULTS3.1 FAILURE MODESBefore cracking, all the strengthened specimens exhibited bending behavior similar to the unstrengthened specimen. This shows that the CFRP reinforcement is unable to contribute to the increase of the stiffness and strength in the elastic domain. However, after cracking, thebending stiffness and strength of the strengthened specimens were seen to increase significantly until failure compared to the unstrengthened specimens.Examining the final failure, the unstrengthened control specimen presented typical bending failure mode which proceeds by the yielding of steel reinforcement followed by compression failure of concrete. The failure of CPL-50-BOND, EBR specimen, began with the separation of CFRP reinforcement and concrete at mid-span to exhibit finally brittle debonding failure (Figure 3). Failure of NSM-PL-15, NSM specimen, occurred with the rupture of the FRP reinforcement. Failure of the remaining NSM specimens(NSM-PL-25, NSM-PL25*2, and NSM-PL-2S) occurred through the simultaneous separation of the CFRP reinforcement and epoxy from concrete (Figure 4, 5, and 6).Table 3 summarizes the failure modes.3.2 STRENGTHENING EFFECTFigure 7 ploted the load-deflection curves of EBR and NSM specimens. The specimens with EBR,CPL-50-BOND, presented ultimate load increased by 30% compared to the unstrengthened specimen, while NSM specimens (NSM-PL-15, NSM-PL-25) increased the ultimate load by 40 to 53%.Observation of Figure 7 reveals that even if CPL-50-BOND with relatively large cross-sectional areaof CFRP reinforcement developed larger initial stiffness, premature debonding failure occurred because its bonding area is much smaller than NSM-PL-15, NSM-PL-25. EBR specimen behaved similarly to the unstrengthened control specimen after debonding failure. In Figure 7, the stiffness of NSM specimens before yielding of steel reinforcement was smaller than the stiffness developed by EBR specimen because NSM specimens have the smaller cross-sectional area of CFRP reinforcement than EBR specimen. The ultimate load and yield load are seen to increasewith the cross-sectional area of NSM reinforcement.Examining the ultimate strain of FRP summarized in Table 3, the maximum strain for EBR specimenappears to attain 30% of the ultimate strain, and 80 to 100% for NSM specimens. This proves that the NSM system is utilizing CFRP reinforcement efficiently(2S with the same cross-sectional area as CPL-50-Bond resented ultimate load increased by 95%, 90% compared to the unstrengthened specimen,respectively. Considering the same cross-sectional area, the strengthening effect of NSM specimens issuperior to the EBR specimen.In Figure 8,NSM-PL-25*2 and NSM-PL-2S, NSM specimens with space variables,showed that the strengthening effect of the specimen with narrow spaceis slightly increased by 2.5%as compared to the specimen with wide space.4. CONCLUSIONSPerformance tests have been carried out on RC beams strengthened with NSM systems. The followingconclusions were derived from the experimental results.It has been seen that NSM specimens utilized the CFRP reinforcement more efficiently than the EBR specimen. According to the static loading test results, the strengthening performances were improvedin NSM specimens compared with EBR specimen. However, the specimens NSM-PL-25, NSM-PL-25*2 and NSM-PL-2S failed by the separation of the CFRP reinforcements and epoxy adhesive from the concrete. Consequently, it is necessary to take somecountermeasures to prevent debonding failure for NSM specimens.Considering the same cross-sectional area, the strengthening effect of NSM specimens is superior to EBR specimen. NSM-PL-25*2 and NSM-PL-2S, NSM specimens with space variables, showed that the strengthening effect ofthe specimen with narrow space is slightly increased as compared to the specimen with wide space.5. REFERENCES1. Hassan, T. and Rizkalla, S. (2003), Investigation of Bond in Concrete Structures Strengthenedwith Near Surface Mounted Carbon Fiber Reinforced Polymer Strips”, Journal of Composites for Construction, Vol 7, No. 3, pp. 248-2572. Täljsten, B. and Carolin, A. (2001), “Concrete Beams Strengthened with Near Surface MountedCFRP Laminates”, Proceeding of the fifth international conference of ibre-reinforced plastics forreinforced concrete structures (FRPRCS-5), Cambridge, UK, 16-18 July 2001, pp. 107-1163. Mirmiran, A., Shahawy, M., Nanni, A., and Karbhari, V. (2004), “Bonded Repair and Retrofit ofConcrete Structures Using FRP Composites”, Recommended Construction Specifications andProcess Control Manual, NCHRP Report 514, Transportation Research Board4. Rizkalla, S., and Hassan, T. (2002), “Effectiveness of FRP for Strengthening Concrete Bridges”,Structural Engineering International, Vol. 12, No. 2, pp. 89-95近表面埋置加固的钢筋混凝土梁抗弯性能实验研究Woo-Tai JUNG1, Young-Hwan PARK2, Jong-Sup PARK3摘要:本研究介绍了近表面贴埋置加固钢筋混凝土(RC)实验结果。
钢筋混凝土梁在高温下的性能研究
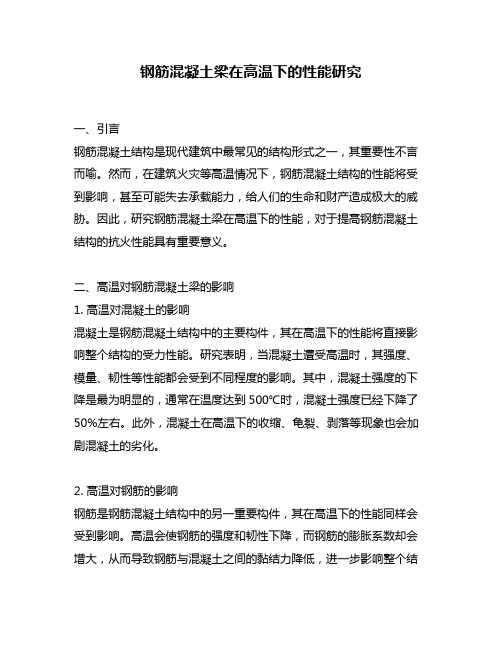
钢筋混凝土梁在高温下的性能研究一、引言钢筋混凝土结构是现代建筑中最常见的结构形式之一,其重要性不言而喻。
然而,在建筑火灾等高温情况下,钢筋混凝土结构的性能将受到影响,甚至可能失去承载能力,给人们的生命和财产造成极大的威胁。
因此,研究钢筋混凝土梁在高温下的性能,对于提高钢筋混凝土结构的抗火性能具有重要意义。
二、高温对钢筋混凝土梁的影响1. 高温对混凝土的影响混凝土是钢筋混凝土结构中的主要构件,其在高温下的性能将直接影响整个结构的受力性能。
研究表明,当混凝土遭受高温时,其强度、模量、韧性等性能都会受到不同程度的影响。
其中,混凝土强度的下降是最为明显的,通常在温度达到500℃时,混凝土强度已经下降了50%左右。
此外,混凝土在高温下的收缩、龟裂、剥落等现象也会加剧混凝土的劣化。
2. 高温对钢筋的影响钢筋是钢筋混凝土结构中的另一重要构件,其在高温下的性能同样会受到影响。
高温会使钢筋的强度和韧性下降,而钢筋的膨胀系数却会增大,从而导致钢筋与混凝土之间的黏结力降低,进一步影响整个结构的受力性能。
3. 高温对梁的影响钢筋混凝土梁是钢筋混凝土结构中常用的承载构件之一,其在高温下的性能也必须引起重视。
高温将会导致梁的刚度和强度下降,而且还可能会产生裂缝和变形。
此外,高温下的混凝土龟裂、剥落等现象也会严重影响梁的受力性能。
三、研究方法1. 试验方法为了研究钢筋混凝土梁在高温下的性能,通常采用试验方法进行研究。
试验通常分为两类:一是对整个钢筋混凝土梁进行高温热模拟试验,模拟真实火灾的高温条件,研究梁在高温下的受力性能;二是对混凝土和钢筋分别进行高温试验,研究其在高温下的性能变化规律。
2. 数值模拟方法数值模拟方法是一种较为常用的研究方法,其主要通过有限元分析等方法,模拟钢筋混凝土梁在高温下的受力性能。
数值模拟方法具有试验方法无法达到的优点,如可以对多种参数进行研究,同时可以模拟不同的温度、载荷等条件,具有较好的灵活性和实用性。
钢筋混凝土中英文对照外文翻译文献

中英文资料对照外文翻译目录1 中文翻译 (1)1.1钢筋混凝土 (1)1.2土方工程 (2)1.3结构的安全度 (3)2 外文翻译 (6)2.1 Reinforced Concrete (6)2.2 Earthwork (7)2.3 Safety of Structures (9)1 中文翻译1.1钢筋混凝土素混凝土是由水泥、水、细骨料、粗骨料(碎石或;卵石)、空气,通常还有其他外加剂等经过凝固硬化而成。
将可塑的混凝土拌合物注入到模板内,并将其捣实,然后进行养护,以加速水泥与水的水化反应,最后获得硬化的混凝土。
其最终制成品具有较高的抗压强度和较低的抗拉强度。
其抗拉强度约为抗压强度的十分之一。
因此,截面的受拉区必须配置抗拉钢筋和抗剪钢筋以增加钢筋混凝土构件中较弱的受拉区的强度。
由于钢筋混凝土截面在均质性上与标准的木材或钢的截面存在着差异,因此,需要对结构设计的基本原理进行修改。
将钢筋混凝土这种非均质截面的两种组成部分按一定比例适当布置,可以最好的利用这两种材料。
这一要求是可以达到的。
因混凝土由配料搅拌成湿拌合物,经过振捣并凝固硬化,可以做成任何一种需要的形状。
如果拌制混凝土的各种材料配合比恰当,则混凝土制成品的强度较高,经久耐用,配置钢筋后,可以作为任何结构体系的主要构件。
浇筑混凝土所需要的技术取决于即将浇筑的构件类型,诸如:柱、梁、墙、板、基础,大体积混凝土水坝或者继续延长已浇筑完毕并且已经凝固的混凝土等。
对于梁、柱、墙等构件,当模板清理干净后应该在其上涂油,钢筋表面的锈及其他有害物质也应该被清除干净。
浇筑基础前,应将坑底土夯实并用水浸湿6英寸,以免土壤从新浇的混凝土中吸收水分。
一般情况下,除使用混凝土泵浇筑外,混凝土都应在水平方向分层浇筑,并使用插入式或表面式高频电动振捣器捣实。
必须记住,过分的振捣将导致骨料离析和混凝土泌浆等现象,因而是有害的。
水泥的水化作用发生在有水分存在,而且气温在50°F以上的条件下。
浅谈高温作用下钢筋混凝土材料性能
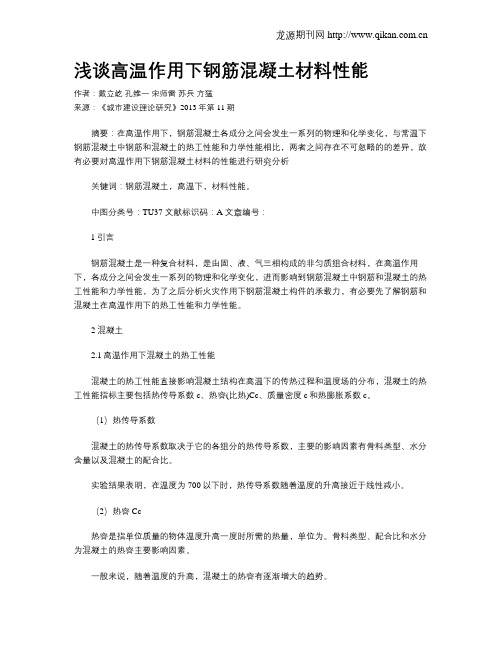
浅谈高温作用下钢筋混凝土材料性能作者:戴立屹孔维一宋师雷苏兵方猛来源:《城市建设理论研究》2013年第11期摘要:在高温作用下,钢筋混凝土各成分之间会发生一系列的物理和化学变化,与常温下钢筋混凝土中钢筋和混凝土的热工性能和力学性能相比,两者之间存在不可忽略的的差异,故有必要对高温作用下钢筋混凝土材料的性能进行研究分析关键词:钢筋混凝土,高温下,材料性能。
中图分类号:TU37 文献标识码:A 文章编号:1引言钢筋混凝土是一种复合材料,是由固、液、气三相构成的非匀质组合材料,在高温作用下,各成分之间会发生一系列的物理和化学变化,进而影响到钢筋混凝土中钢筋和混凝土的热工性能和力学性能,为了之后分析火灾作用下钢筋混凝土构件的承载力,有必要先了解钢筋和混凝土在高温作用下的热工性能和力学性能。
2 混凝土2.1高温作用下混凝土的热工性能混凝土的热工性能直接影响混凝土结构在高温下的传热过程和温度场的分布,混凝土的热工性能指标主要包括热传导系数c、热容(比热)Cc、质量密度c和热膨胀系数c。
(1)热传导系数混凝土的热传导系数取决于它的各组分的热传导系数,主要的影响因素有骨料类型、水分含量以及混凝土的配合比。
实验结果表明,在温度为700以下时,热传导系数随着温度的升高接近于线性减小。
(2)热容 Cc热容是指单位质量的物体温度升高一度时所需的热量,单位为。
骨料类型、配合比和水分为混凝土的热容主要影响因素。
一般来说,随着温度的升高,混凝土的热容有逐渐增大的趋势。
(3)质量密度 c ()质量密度c是指单位体积的物体的质量,单位。
混凝土构件一般长时间放置在自然环境中,水分蒸发较快,可以忽略水分对混凝土质量密度的影响。
可以将c看作常数2400 。
(4)热膨胀系数 c混凝土的热膨胀系数是指温度升高一度时物体单位长度的伸长量,单位是。
混凝土为热惰性材料,在同一个截面上,混凝土构件的温度由表面到内部分布不均匀,存在温差,而且沿混凝土试件的轴线方向,温度分布也不均匀,因此由温度产生的膨胀变形并不是均匀的,试件的实际伸长量是平均膨胀变形的结果。
钢筋混凝土构件受高温作用后的力学性能研究
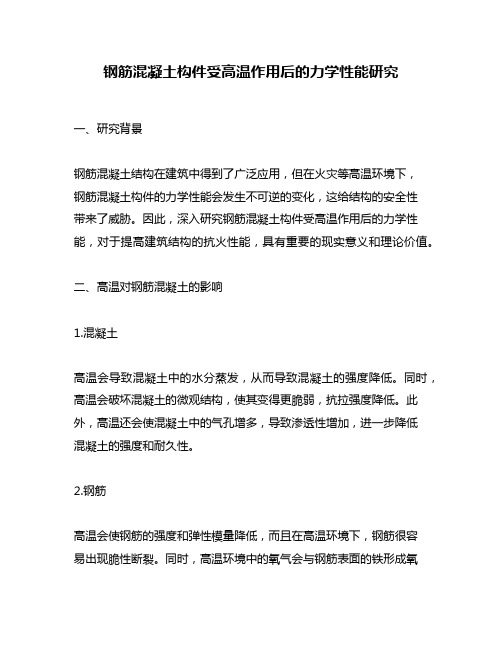
钢筋混凝土构件受高温作用后的力学性能研究一、研究背景钢筋混凝土结构在建筑中得到了广泛应用,但在火灾等高温环境下,钢筋混凝土构件的力学性能会发生不可逆的变化,这给结构的安全性带来了威胁。
因此,深入研究钢筋混凝土构件受高温作用后的力学性能,对于提高建筑结构的抗火性能,具有重要的现实意义和理论价值。
二、高温对钢筋混凝土的影响1.混凝土高温会导致混凝土中的水分蒸发,从而导致混凝土的强度降低。
同时,高温会破坏混凝土的微观结构,使其变得更脆弱,抗拉强度降低。
此外,高温还会使混凝土中的气孔增多,导致渗透性增加,进一步降低混凝土的强度和耐久性。
2.钢筋高温会使钢筋的强度和弹性模量降低,而且在高温环境下,钢筋很容易出现脆性断裂。
同时,高温环境中的氧气会与钢筋表面的铁形成氧化层,使钢筋的锈蚀速度加快。
三、钢筋混凝土受高温作用后的力学性能研究方法1.试验方法钢筋混凝土构件受高温作用后的力学性能研究,需要通过试验来进行。
试验通常采用恒温炉对混凝土构件进行高温处理,然后对处理后的构件进行力学性能试验。
2.试验内容试验内容包括构件的强度、变形和破坏形态等方面的研究。
其中,强度研究包括混凝土的抗压强度和钢筋的屈服强度;变形研究包括混凝土和钢筋的变形,并通过变形试验来研究其变形特性;破坏形态研究则是通过观察试件的破坏形态来了解其破坏机理。
四、钢筋混凝土受高温作用后的力学性能研究结果1.强度钢筋混凝土构件在高温作用下,其抗压强度和屈服强度均会明显降低,而且降低的幅度随着温度的升高而增大。
同时,钢筋混凝土构件的强度下降速度也随着高温时间的延长而增大。
2.变形钢筋混凝土构件在高温作用下,其变形特性也会发生明显变化。
混凝土的变形增大,而且在高温作用后,混凝土的变形能力下降,易出现裂缝。
钢筋的变形也会增加,但相对于混凝土,钢筋的变形能力下降的幅度要小。
3.破坏形态钢筋混凝土构件在高温作用下,其破坏形态也会发生变化。
在低温下,构件的破坏主要是混凝土的压碎破坏和钢筋的屈曲破坏,而在高温下,构件的破坏主要是混凝土的开裂破坏和钢筋的脆性断裂。
土木工程外文文献翻译
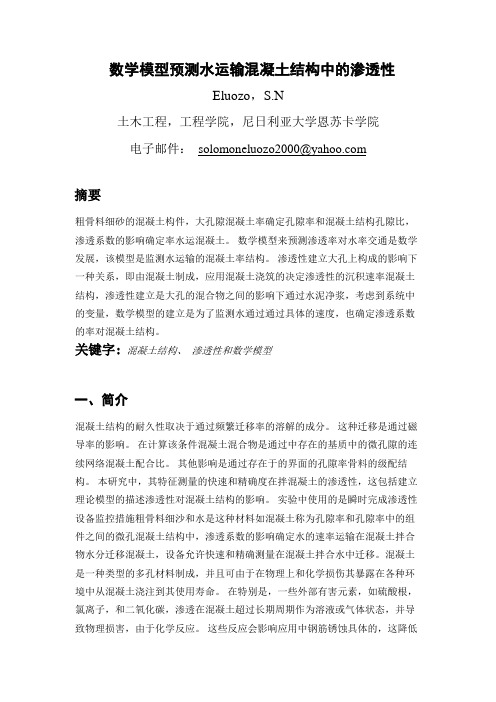
数学模型预测水运输混凝土结构中的渗透性Eluozo,S.N土木工程,工程学院,尼日利亚大学恩苏卡学院电子邮件:solomoneluozo2000@摘要粗骨料细砂的混凝土构件,大孔隙混凝土率确定孔隙率和混凝土结构孔隙比,渗透系数的影响确定率水运混凝土。
数学模型来预测渗透率对水率交通是数学发展,该模型是监测水运输的混凝土率结构。
渗透性建立大孔上构成的影响下一种关系,即由混凝土制成,应用混凝土浇筑的决定渗透性的沉积速率混凝土结构,渗透性建立是大孔的混合物之间的影响下通过水泥净浆,考虑到系统中的变量,数学模型的建立是为了监测水通过通过具体的速度,也确定渗透系数的率对混凝土结构。
关键字:混凝土结构、渗透性和数学模型一、简介混凝土结构的耐久性取决于通过频繁迁移率的溶解的成分。
这种迁移是通过磁导率的影响。
在计算该条件混凝土混合物是通过中存在的基质中的微孔隙的连续网络混凝土配合比。
其他影响是通过存在于的界面的孔隙率骨料的级配结构。
本研究中,其特征测量的快速和精确度在拌混凝土的渗透性,这包括建立理论模型的描述渗透性对混凝土结构的影响。
实验中使用的是瞬时完成渗透性设备监控措施粗骨料细沙和水是这种材料如混凝土称为孔隙率和孔隙率中的组件之间的微孔混凝土结构中,渗透系数的影响确定水的速率运输在混凝土拌合物水分迁移混凝土,设备允许快速和精确测量在混凝土拌合水中迁移。
混凝土是一种类型的多孔材料制成,并且可由于在物理上和化学损伤其暴露在各种环境中从混凝土浇注到其使用寿命。
在特别是,一些外部有害元素,如硫酸根,氯离子,和二氧化碳,渗透在混凝土超过长期周期作为溶液或气体状态,并导致物理损害,由于化学反应。
这些反应会影响应用中钢筋锈蚀具体的,这降低了耐久寿命,例如钢筋和力量。
因此,它是非常重要的是插入腐蚀抑制剂为在超过临界恶化元件的情况下钢棒腐蚀的钢筋的位置量[1]。
然而,这是非常困难的保证在使用该应用传统技术钢筋位置的耐腐蚀性腐蚀抑制剂仅在混凝土[2-3]的表面上。
土木工程专业钢筋混凝土结构抗震性能外文翻译文献

文献信息:文献标题:Seismic Performance of Reinforced Concrete Buildings with Masonry Infill(砌体填充钢筋混凝土建筑的抗震性能研究)文献作者:Girma Zewdie Tsige,Adil Zekaria文献出处:《American Journal of Civil Engineering》,2018,6(1):24-33 字数统计:英文3088单词,16137字符;中文4799汉字外文文献:Seismic Performance of Reinforced Concrete Buildings withMasonry InfillAbstract Unreinforced masonry Infills modify the behavior of framed structures under lateral loads; however, in practice, the infill stiffness is commonly ignored in frame analysis, resulting in an under-estimation of stiffness and natural frequency. The structural effect of hollow concrete block infill is generally not considered in the design of columns as well as other structural components of RC frame structures. The hollow concrete block walls have significant in-plane stiffness contributing to the stiffness of the frame against lateral load. The scope of present work was to study seismic performance of reinforced concrete buildings with masonry infill in medium rise building. The office medium rise building is analyzed for earthquake force by considering three type of structural system. i.e. Bare Frame system, partially-infilled and fully- Infilled frame system. Effectiveness of masonry wall has been studied with the help of five different models. Infills were modeled using the equivalent strut approach. Nonlinear static analyses for lateral loads were performed by using standard package ETABS, 2015 software. The comparison of these models for different earthquake response parameters like base shear vs roof displacement, Story displacement, Story shear and member forces are carried out. It is observed that the seismic demand in the bare frame is significantly large when infillstiffness is not considered, with larger displacements. This effect, however, is not found to be significant in the infilled frame systems. The results are described in detail in this paper.Keywords: Bare Frame, Infilled Frame, Equivalent Diagonal Strut, Infill, Plastic Hinge1.IntroductionInfill have been generally considered as non-structural elements, although there are codes such as the Eurocode-8 that include rather detailed procedures for designing infilled R/C frames, presence of infill has been ignored in most of the current seismic codes except their weight. However, even though they are considered non-structural elements the presence of infill in the reinforced concrete frames can substantially change the seismic response of buildings in certain cases producing undesirable effects (tensional effects, dangerous collapse mechanisms, soft story, variations in the vibration period, etc.) or favorable effects of increasing the seismic resistance capacity of the building.The present practice of structural analysis is also to treat the masonry infill as non- structural element and the analysis as well as design is carried out by only using the mass but neglecting the strength and stiffness contribution of infill. Therefore, the entire lateral load is assumed to be resisted by the frame only.Contrary to common practice, the presence of masonry infill influence the over- all behavior of structures when subjected to lateral forces. When masonry infill are considered to interact with their surrounding frames, the lateral stiffness and the lateral load capacity of the structure largely increase.The recent advent of structural design for a particular level of earthquake performance, such as immediate post-earthquake occupancy, (termed performance based earthquake engineering), has resulted in guidelines such as ATC-40 (1996) FEMA-273 (1996) and FEMA-356 (2000) and standards such as ASCE-41 (2006), among others. The different types of analyses described in these documents, pushover analysis comes forward because of its optimal accuracy, efficiency and ease of use.The infill may be integral or non-integral depending on the connectivity of the infill to the frame. In the case of buildings under consideration, integral connection is assumed. The composite behavior of an infilled frame imparts lateral stiffness and strength to the building. The typical behavior of an infilled frame subjected to lateral load is illustrated in Figures 1 (a) and (b).Figure1. Behavior of infilled frames (Govindan, 1986).In this present paper five models of office building with different configuration of masonry infill are generated with the help of ETABS 2015 and effectiveness has been checked. Pushover analysis is adopted for the evaluation of the seismic response of the frames. Each frame is subjected to pushover loading case along negative X-direction.2.Building DescriptionMulti-storey rigid jointed frame mixed use building G+9 (Figure 2), was selected in the seismic zone (Zone IV) of Ethiopia and designed based on the Ethiopian Building Code Standard ESEN: 2015 and European Code-2005. ETABS 2015 was used for the analysis and design of the building by modeling as a 3-D space frame system.Figure 2. Typical building plan.Seismic performance is predicted by using performance based analysis of simulation models of bare and infilled non ductile RC frame buildings with different arrangement of masonry wall. The structure will be assumed to be new, with no existing infill damage.Building Data:1.Type of structure = Multi-storey rigid jointed frameyout = as shown in figure 23.Zone = Iv4.Importance Factor = 15.Soil Condition = hard6.Number of stories = Ten (G+9)7.Height of Building =30 m8.Floor to floor height = 3 m9.External wall thickness =20cm10.Internal wall thickness=15cm11.Depth of the floor slab =15cm12.depth of roof slab=12cm13.Size of all columns = 70×70cm14.Size of all beams = 70 × 40cm15.Door opening size=100×200cm16.Window opening size =200×120cm3.Structural Modeling and AnalysisTo understand the effect of masonry wall in reinforced concrete frame, with a total of five models are developed and pushover analysis has been made in standard computer program ETABS2015. In this particular study pushover loading case along negative X-axis is considered to study seismic performance of all models. Since the out of plane effect is not studied in this paper, only the equivalent strut along X-axis are considered to study the in plane effect and masonry walls along Y-axis are not considered in all models. From this different condition, all models are identified by their names which are given below.3.1.Different Arrangement of the Building ModelsTo understand the effect of masonry wall in reinforced concrete frame, with a total of five models are developed and pushover analysis has been made in standard computer program ETABS2015. In this particular study pushover loading case along negative X-axis is considered to study seismic performance of all models.Model 1:- Bare reinforced concrete frame: masonry infill walls are removed from the building along all storiesModel 2:-Reinforced concrete frame with 75% of masonry wall removed from fully infilled frameFigure 3. Plan View Model 2.Model 3:- Reinforced concrete frame with half of of masonry wall removed from fully infilled frameModel 4:- Reinforced concrete frame with 25% of masonry wall removed from fully infilled frameFigure 5. Plan view of Model 4.Model 5:- Fully infilled reinforced concrete frame (Base frame)3.2.Modeling of Masonry InfillIn the case of an infill wall located in a lateral load resisting frame the stiffness and strength contribution of the infill are considered by modelling the infill as an equivalent compression strut (Smith).Because of its simplicity, several investigators have recommended the equivalent strut concept. In the present analysis, a trussed frame model is considered. This type of model does not neglect the bending moment in beams and columns. Rigid joints connect the beams and columns, but pin joints at the beam-to-column Junctions connect the equivalent struts.Infill parameters (effective width, elastic modulus and strength) are calculated using the method recommended by Smith. The length of the strut is given by the diagonal distance D of the panel (Figure 7) and its thickness is given by the thickness of the infill wall. The estimation of width w of the strut is given below. The initial elastic modulus of the strut Ei is equated to Em the elastic modulus of masonry. As per UBC (1997), Em is given as 750fm, where fm is the compressive stress of masonry in MPa. The effective width was found to depend on the relative stiffness of the infill to the frame, the magnitude of the diagonal load and the aspect ratio of the infilled panel.Figure 7. Strut geometry (Ghassan Al-Chaar).The equivalent strut width, a, depends on the relative flexural stiffness of the infill to that of the columns of the confining frame. The relative infill to frame stiffness shall be evaluated using equation 1 (Stafford-Smith and Carter 1969):Using this expression, Mainstone (1971) considers the relative infill to frame flexibility in the evaluation of the equivalent strut width of the panel as shown in equation 2.Where:λ1= Relatire infill to frame stiffness garameterα= Equivalent width of infill strut, cmE m = modulus of elasticity of masonry infill, MPaE c = modulus of elasticity of confining frame, MPaI column = moment of inertia of masonry infill, cm4t = Gross thickness of the infill, cmh = height of the infill panel, cmθ = Angle of the concentric equivalent strut, radiansD = Diagonal length of infill, cmH = Height of the confining frame, cm3.3.Eccentricity of Equivalent StrutThe equivalent masonry strut is to be connected to the frame members as depicted in Figure 8. The infill forces are assumed to be mainly resisted by the columns, and the struts are placed accordingly. The strut should be pin-connected to the column at a distance l column from the face of the beam. This distance is defined in Equations 3 and 4 and is calculated using the strut width, a.Figure 8. Placement of strut (Ghassan Al-Chaar).3.4.Plastic Hinge PlacementPlastic hinges in columns should capture the interaction between axial load and moment capacity. These hinges should be located at a minimum distance l column from the face of the beam as shown in figure 9. Hinges in beams need only characterize the flexural behavior of the member.Figure 9. Plastic hinge placement (Ghassan Al-Chaar).3.5. Analysis of the Building ModelsThe non-structural elements and components that do not significantly influence the building behavior were not modeled. The floor slabs are assumed to act as diaphragms, which ensure integral action of all the vertical lateral load-resisting elements. Beams and columns were modeled as frame elements with the centerlines joined at nodes. Rigid offsets were provided from the nodes to the faces of the columns or beams. The stiffness for columns and beams were taken as 0.7EcIg, 0.35EcIg respectively accounting for the cracking in the members and the contribution of flanges in the beams.The weight of the slab was distributed to the surrounding beams as per ESEN1992:2015. The mass of the slab was lumped at the Centre of mass location at each floor level. This was located at the design eccentricity from the calculated centre of stiffness. Design lateral forces at each storey level were applied at the Centre of mass locations independently in two horizontal directions (X- and Y- directions).Staircases and water tanks were not modeled for their stiffness but their masses were considered in the static and dynamic analyses. The design spectrum for hard soil as specified in ESEN1998:2015 was used for the analysis.The effect of soil-structure interaction was ignored in the analyses. The columns were assumed to be fixed at the level of the bottom of the base slabs of respective isolated footings.Figure 10. Force-Deformation Relation for Plastic Hinge in Pushover Analysis (Habibullah. et al.,1998).4.Analysis Results and DiscussionsThe results of pushover analysis of reinforced concrete frame with different configuration of masonry wall are presented. Analysis of the models under the static and dynamic loads has been performed using Etabs 2015 software. All required data are provided in software and analyzed for total five models to get the result in terms of Base shear vs monitored roof displacement, Storey shear, story displacement and Element force. Subsequently these results are compared for reinforced concrete frame with different configuration of masonry wall.4.1.Base Shear vs Monitored Roof Displacement CurveBased up on the Displacement coefficient method of ASCE 41-13 all the five building models are analyzed in ETABS 2015 standard structural software and the static pushover curve is generated as shown in figure 11.Figure 11. Pushover analysis result for 10-story RC building.The presence of the infill wall both strengthens and stiffens the system, as illustrated in figure 11. For the case study building, the fully-infilled frame has approximately 3 times larger intial stiffness and 1.5 times greater peak strength than the bare frame. In figure 11, the first drop in strength for the fully and partially-infilled frame is due to the brittle failure of masonry materials initiating in the first-story infill walls. This behavior after first-story wall failure is due towall-frame interaction and depends on the relative strength of the infill and framing.So, based on these results, infill walls can be beneficial as long as they are properly taken into consideration in the design process and the failure mechanism is controlled.4.2.Story Displacement for Different ModelsFigure 12. shows the comparative study of seismic demand in terms of lateral story displacement amongst all the five types of reinforced concrete frame with different configuration of infill. The lateral displacement obtained from the bare frame model is the maximum which is about 60% greater than that of fully infilled frame, nearly 50% greater than that of frame with 25% of the masonry wall reduced, about 40% greater than that of frame with 50% of the masonry wall reduced and 30% greater than that of frame with 75% of the masonry wall reduced.Figure 12. Comparison of Story displacements for different models.Thus, the infill panel reduces the seismic demand of reinforced concrete buildings. The lateral story displacement is dramatically reduced due to introduction of infill. This probably is the cause of building designed in conventional way behaving near elastically even during strong earthquake.4.3.Member ForcesIn this project to understand the effect of different configuration of infill in reinforced concrete frame; study of the behavior of the column in all models for axialloads was conducted. Total of five nonlinear models are analyzed in ETABS 2015 and all models have same plan of building, therefore the position and label of columns are same in all plans of models which is shown in figure 2. After analysis consider the column no. 1(C1) shown in figure 2. from all models for pushover load case and get the axial forces of column at performance point at every story from software, which is given in table 1 and the values for each model is compared with the bare frame model.Table 1. Comparison of axial force for different models. (KN)From this observation, it is evident that when an infilled frame is loaded laterally, the columns take the majority of the force and shear force exerted on the frame by the infill which is modeled as the eccentric equivalent struts. Generally, the relative increase of axial force is observed when the percentage of infill in reinforced concrete frame increases. It is observed that fully infilled reinforced concrete frame showed around 10% increase in axial force relative to bare frame model. The other infill models showed a lesser increase. The effect of infill on columns is to increase the shear force and to reduce bending moments.In general compared to bare frame model, the infilled models predicted higher axial and shear forces in columns but lower bending moments in both beams and columns. Thus, the effect of infill panel is to change the predominantly a frame action of a moment resisting frame system towards truss action.4.4.Story ShearStory shear is the total horizontal seismic shear force at the base of structure. Results from static pushover analysis at performance point for the case study buildings are shown in figure 13.Figure 13. Comparison of story shear for different model.As observed from the figure 13 the story shear calculated on the basis of bare frame model gave a lesser value than the other infilled frames; It was observed that the story shear in fully infilled frame is nearly 15% greater compared to bare frame model and frame with 25% of the masonry wall reduced was nearly 10% greater compared to the bare frame, frame with 50% of the masonry wall reduced is nearly 8% greater compared to the bare frame and frame with 75% of the masonry wall reduced is about 5% greater compared to the bare frame.Since the bare frame models do not take in to account the stiffness rendered by the infill panel, it gives significantly longer time period. And hence smaller lateral forces. And when the infill is modeled, the structure becomes much stiffer than the bare frame model. Therefore, it has been found that calculation of earthquake forces by treating RC frames as ordinary frames without regards to infill leads to underestimation of base shear. This is because of bare frame is having larger value of fundamental natural time period as compared to other models due to absence of masonry infill walls. Fundamental natural period get increased and therefore base shear get reduced.5.ConclusionsFrom above results it is clear that pushover curve show an increase in initial stiffness, strength, and energy dissipation of the infilled frame, compared to the bareframe, despite the wall’s brittle failure modes.Due to the introduction of infill the displacement capacity decreases as depicted from the displacement profile (Figure 12). The lateral displacement obtained from the bare frame model is the maximum which is about 60% greater than that of infilled frame.The presence of masonry walls is to change a frame action of a moment resisting frame structure towards a truss action. When infills are present, shear and axial force demands are considerably higher leaving the beam or column vulnerable to shear failure. The axial force and shear force of the bare frame is less than that of the infilled frame. Columns take the majority of the forces exerted on the frame by the infill because the eccentrically modeled equivalent struts transfers the axial load and shear force transferred from the action of lateral loads directly to the columns.The story shear calculated on the basis of bare frame model gave a lesser value than the other infilled frames. It was observed that fully infilled frame is nearly 15% greater compared to bare frame model; frame with 25% of the masonry wall reduced was nearly 10% greater compared to the bare frame; frame with 50% of the masonry wall reduced is nearly 8% greater compared to the bare frame and frame with 75% of the masonry wall reduced is about 5% greater compared to the bare frame. This is because the bare frame models do not takes in to account the stiffness rendered by the infill panel, it gives significantly longer time period.中文译文:砌体填充钢筋混凝土建筑的抗震性能研究摘要无配筋砌体填充对框架结构在侧向荷载作用下的受力性能有很大的影响,但在实际应用中,往往忽略了框架结构的填充刚度,导致对框架结构的刚度和固有频率的估计不足。
- 1、下载文档前请自行甄别文档内容的完整性,平台不提供额外的编辑、内容补充、找答案等附加服务。
- 2、"仅部分预览"的文档,不可在线预览部分如存在完整性等问题,可反馈申请退款(可完整预览的文档不适用该条件!)。
- 3、如文档侵犯您的权益,请联系客服反馈,我们会尽快为您处理(人工客服工作时间:9:00-18:30)。
土木外文翻译--高温下钢筋混凝土中钢筋的性能外文原文:Research Letters in Materials ScienceVolume 2008 (2008), Article ID 814137, 4 pagesdoi:10.1155/2008/814137Research LetterProperties of Reinforced Concrete Steel Rebars Exposed to High Temperaturesİlker Bekir Topçu and Cenk KarakurtDepartment of Civil Engineering, Eskişehir Osmangazi University, 26480 Eskişehir, TurkeyReceived 12 February 2008; Accepted 31 March 2008Academic Editor: Rajiv S. MishraCopyright © 2008 İlker Bekir Topçu and Cenk Karakurt. This is an open access article distributed under the Creative Commons Attribution License, which permits unrestricted use, distribution, and reproduction in any medium, provided the original work is properly cited.The deterioration of the mechanical properties of yield strength and modulus of elasticity is considered as the primary element affecting the performance of steel structures under fire. In this study, hot-rolled S220 and S420 reinforcement steel rebars were subjected to high temperatures to investigate the fire performance of these materials. It is aimed to determine the remaining mechanical properties of steel rebars after elevated temperatures. Steels were subjected to 20, 100, 200, 300, 500, 800, and 9 5 0 ∘ C temperatures for 3 hours and tensile tests were carried out. Effect of temperature on mechanical behavior of S220 and S420 were determined. All mechanical properties were reduced due to the temperature increase of the steel rebars. It is seen that mechanical properties of S420 steel was influenced more than S220 steel at elevated temperatures.Fire remains one of the serious potential risks to most buildings and structures. Since concrete is widely used in construction, research on fire resistance of concrete becomes more and more important. Many researchers all over the world have done some researches on this subject. The mechanical properties of all common building materials decrease with elevation of temperature. The behavior of a reinforced concrete structure in fire conditions is governed by the properties of the constituent materials, concrete, and steel, at high temperature. Both concrete and steel undergo considerable change in their strength, physical properties, and stiffness by the effects of heating, and some of these changes are not recoverable after subsequent cooling [1].It is necessary to have safe, economical, and easily applicable design methods for steel members subjected to fire. However, without fire protection, steel structures may suffer serious damage or even collapse in a fire catastrophe. This is because the mechanical properties of steel deteriorate by heat during fires, and the yield strength of conventional steel at 600°C is less than 1/3 of the specified yield strength at room temperature [2]. Therefore, conventional steels normally require fire-resistant coating to be applied [3]. The temperature increase in the steel member is governed by the principles of heat transfer. Consequently, it must be recognized that the temperature of the steel member(s) will not usually be the same as the fire temperature in a compartment or in the exterior flame plume.Protected steel will experience a much slower temperature rise during a fire exposure than unprotected steel. Also, fire effect on steel member is influenced with its distance from the center of the fire, and if more ventilation occurs near the steel in a fuel-controlled condition, wherein the ventilation helps to cool the steel by dissipating heat to the surrounding environment [4].Especially, temperature increase of steel and concrete in composite steel-concrete elements leads to a decrease of mechanical properties such as yield stress, Young's modulus, and ultimate compressive strength of concrete [5]. Thus, load bearing of steel decreases when steel or composite structure is subjected to a fire action. If the duration and the intensity of the fire are large enough, the load bearing resistance can fall to the level of the applied load resulting in the collapse of the structure. However, the failure of the World Trade Centre on 11th September 2001 and, in particular, of building WTC7 alerted the engineering profession to the possibility of connection failure under fire conditions [6]. In this study, S220 and S420 ribbed concrete steel rebars were subjected to 7 different temperatures to determine the high temperature behavior of reinforcement steels.Experimental studies were conducted with 10 and 16 mm in diameter and 200 mm in length S220 and S420 reinforcement steel rebars. Test specimens were subjected to 20, 100, 200, 300, 500, 800, and 950°C temperatures in a high furnace for 3 hours, respectively. At the end of the curing process, steels were cooled naturally to the room temperature. Subsequently, tensile tests were applied to steel reinforcement rebars. According to EN 10002-1 tensile strength, yield strength and elongation of the steel rebars were determined for elevated temperatures [7]. The steel specimens tensile strength tests were performed with 60 tons of loading capacity universal tensile strength test machine. The loading speed of the test machine is adjusted according to TS 708 code [8].3. Test Results and Evaluations3.1. Stress-Strain RelationsThe average values for stress-strain relationship for specimens that were exposed to various temperatures are given in Figures 1 and 2. The curves in Figures 1and 2were drawn with the average test results of 10 and 16 mm in diameter steel specimens. The test conditions were meant tosimulate a building that had a fire so the changes in the mechanical properties of reinforcing steels used in structures exposed to high temperature could be determined. As seen from Figure 1, temperatures below 500°C have no significant effect on mechanical properties of preheated and cooled S220 steel rebars. The yield strength and splitting tensile strengths of S220 steels were similar up to this temperature. However, the yield strength and splitting tensile strength of the S220 steel rebars are reducing with the increase of temperatures over 800°C. A similar behavior can be seen from the test results of S420-ribbed steel rebars (Figure 2). All high temperature subjected steel specimens became more ductile temperatures above 800°C.Figure 1: Stress-strain curve of S220 steel rebar.Figure 2: Stress-strain curve of S420-ribbed steel rebar.3.2. Yield StrengthYield strength of both reinforcing steel rebars was affected with the elevated exposure temperatures. It can be concluded from Figure 3that there is no variation in yield strength of reinforcing steels with cover up to 300°C. Plain reinforcing steel rebars have experienced the strain hardening already for this temperature. According to Eurocode and TS EN 1993, before 400°C there is no decrease in yield strength, but after this temperature a significant yield strength loss occurs [9]. The yield strength losses of both S220 and S420 steel rebars were 46% and 84% for 800°C exposure temperature, respectively. For further increase of temperature at 950°C, yield strength decreases were 64% and 89%, respectively. According to these results, the remaining yield strength of S220 steel reinforcing rebar is higher than S420-ribbed steel rebar after high-temperature exposure.Figure 3: Yield strength of steel rebars against temperature.3.3. Tensile StrengthThe tensile strength variation of steel reinforcement rebars exposed to elevated temperatures is given in Figure 4. On the light of these results, there was no significance reducing of tensile strength for both types of steel rebars up to 500°C temperature.The tensile strength losses of both S220 and S420 steel rebars were 51% and 85% for 800°C exposure temperature, respectively. For the highest exposure temperature at 950°C, tensile strength decreases were 60% and 90%, respectively. According to these results, the remaining tensile strength of S220 steel reinforcing rebar is higher than S420-ribbed steel rebar after high-temperature exposure. However, it should be considered that the possibility of complete strength loss of steel rebars at high temperatures when a structure is subjected to a huge fire. The remaining strengths of both reinforcing steel rebars after 500°C are lower than the design strengths of these steels. Consequently, the remaining strength of the steel rebars in structures is influenced with the exposure time and type of fire depending on the heat transfer through concrete cover to steel parts [10].Figure 4: Tensile strength of steel rebars against temperature.3.4. ElongationThe relation between high temperature and splitting elongation ratio can be seen from Figure 5. The figure is demonstrated that both steel rebars show a same elongation behavior under elevated temperatures. The elongation ratios of S220 steel rebars are higher than S420 rebars depending on the ductile fracture behavior of this steel. After a fire inside the reinforced concrete building, the deflections of the structural members increase with the ductile behavior of the steel reinforcement at high temperatures.Figure 5: Elongation ratios of steel rebars against temperature.The elongation ratios were slightly increased up to 300°C, however, above this temperature material becomes brittle with decrease of the elongation values. The elongation losses of both S220 and S420 steel rebars were 1.2% and 1.6% for 800°C exposure temperature, respectively. For further increase of temperature at 950°C, elongation ratios decreases were 1.6% and 3.3%, respectively. According to these results, the elongation capacity of S420 steel is lower than S220 steel under elevated temperatures. The S420 steel showed a brittle fracture behavior under elevated temperatures. This behavior is not sufficient for rebar steel in reinforced concrete structures.3.5. ToughnessThe energy absorbent capacity of materials used in construction should be higher against dynamic earthquake loads. The fracture energy of materials is defined with the toughness concept. The toughness values of the steel rebars used in experimental studies are given in Figure 6. According to test results, the toughness values of both types of steels were decreased after elevated temperature exposure. However, up to 300°C, the toughness values were increased due to the ductile behavior of both steels. The toughness losses of both S220 and S420 steel rebars were 16% and 35% for 800°C exposure temperature, respectively. For further increase of temperature at 950°C, toughness decreases were 82% and 88%, respectively.Figure 6: Toughness of steel rebars against temperature.As described in the previous studies, steel structural members loose strength under elevated temperatures. In this study, the mechanical properties of steel rebars were investigated which exposed to high temperatures and cooled to room temperature. According to test results, the most common reinforcing steel rebar S420 showed a brittle fracture mechanism under elevated temperatures. Splitting yield strength, tensile strength, elongation, and toughness values were low for S220 steel. These results demonstrate that S220 type of steel rebar is less affected than S420 steel under elevated temperatures. The authors suggest that the protective cover thickness should be higher for increasing the fire safety of reinforced concrete members.中文翻译:高温下钢筋混凝土中钢筋的性能摘要处于高温环境的钢结构,屈服强度和弹性模量这两项机械指标的恶化被认为是影响其性能的主要因素。