Distinguishing among Scalar Field Models of Dark Energy
Autodesk Nastran 2023 参考手册说明书
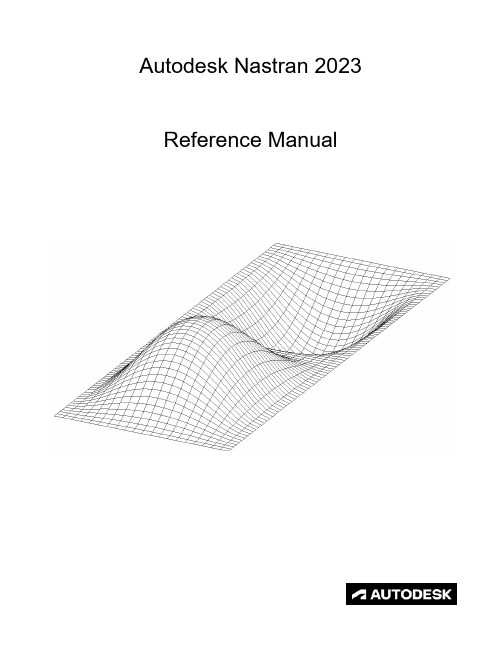
FILESPEC ............................................................................................................................................................ 13
DISPFILE ............................................................................................................................................................. 11
File Management Directives – Output File Specifications: .............................................................................. 5
BULKDATAFILE .................................................................................................................................................... 7
吉他效果器说明书
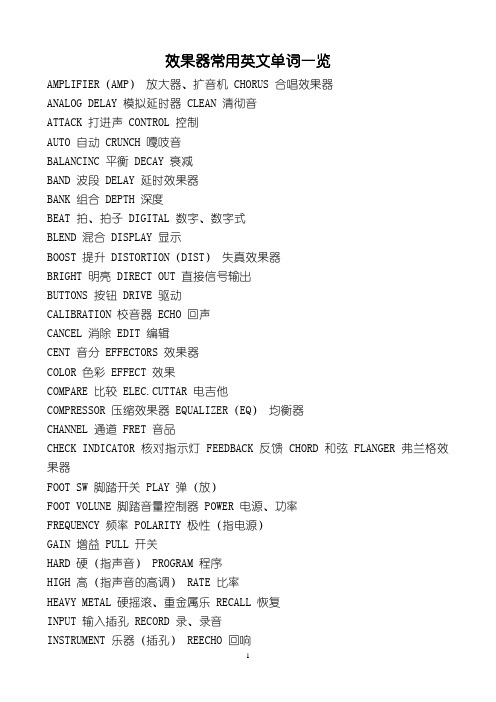
效果器常用英文单词一览AMPLIFIER(AMP)放大器、扩音机 CHORUS 合唱效果器ANALOG DELAY 模拟延时器 CLEAN 清彻音ATTACK 打进声 CONTROL 控制AUTO 自动 CRUNCH 嘎吱音BALANCINC 平衡 DECAY 衰减BAND 波段 DELAY 延时效果器BANK 组合 DEPTH 深度BEAT 拍、拍子 DIGITAL 数字、数字式BLEND 混合 DISPLAY 显示BOOST 提升 DISTORTION(DIST)失真效果器BRIGHT 明亮 DIRECT OUT 直接信号输出BUTTONS 按钮 DRIVE 驱动CALIBRATION 校音器 ECHO 回声CANCEL 消除 EDIT 编辑CENT 音分 EFFECTORS 效果器COLOR 色彩 EFFECT 效果COMPARE 比较 ELEC.CUTTAR 电吉他COMPRESSOR 压缩效果器 EQUALIZER(EQ)均衡器CHANNEL 通道 FRET 音品CHECK INDICATOR 核对指示灯 FEEDBACK 反馈 CHORD 和弦 FLANGER 弗兰格效果器FOOT SW 脚踏开关 PLAY 弹(放)FOOT VOLUNE 脚踏音量控制器 POWER 电源、功率FREQUENCY 频率 POLARITY 极性(指电源)GAIN 增益 PULL 开关HARD 硬(指声音) PROGRAM 程序HIGH 高(指声音的高调) RATE 比率HEAVY METAL 硬摇滚、重金属乐 RECALL 恢复INPUT 输入插孔 RECORD 录、录音INSTRUMENT 乐器(插孔) REECHO 回响JACK 插梢(或指插孔) REPEAT 反复KNOB 旋钮 RESET 重放LAMP 指示灯 REVERB 混响(效果器)LEAD 超载音 RELEASER 解除、释放LIGHT 指示灯 PHYTHM 节奏LEFT 左(声道) RIGHT 右(声道)LEVEL 电平 SEND 发送LOW 低(指音量) SELECTOR 选择钮LONG 长(指持续音) LENSITIVITY 灵敏度LINE OUT 线路输出 STRING 弦线MASTER VOL 总音量 SHORT 短(指持续音)MIDDLE 中音 SHARP 尖锐(指声音)NOISE GATE 噪声闸(效果器的一种)SIGNAL 信号NUT 琴马 SOUND 音响OVER DRIVE 驱动器(效果器的一种)SOFT 柔和(指声音)OUTPUT 输出插孔 SPEED 速度PARAMETRIC EQ 参数均衡器 SPEAKERS 扬声器PHASER 移相(效果器) STAGE TUNER 弦乐校音仪PHONES 耳机 START 启动PICKUPS 拾音器 STOP 停止PITCH 音准 SUSTAIN 持续音(延音)TIME 时间 SWITCH(SW)开关TIMITER 限幅器 SYNTHESIZER 合成器TONE 音调 TEMPO 拍子速度BOOSTER 提升 VOICES 声音TREBLE 高音 VOLUME 音量TUNER 校音 VOLUME PEDAL音量踏板TUBE MANIA 管爆(失真器果的一种)WAW 哇音WRITE 存储(写入)BOSSGT-5 中文说明书第一章:入门一、构造(以GT-3为例,从左到右、由上而下顺序说明)上面板:1、液晶显示屏2、 *** 118;alues调节旋钮(数值轮)3、BANK踏板:GT-3有两个,GT-5一个,用来变换音色组4、脚踏(大踏板、表情踏板、音量踏板)5、设置按钮(多个)6、小踏板:GT-3有4个、GT-5有5个变换小踏板,用来变换某组内的音色号,最右边为1个控制踏板(C踏板)背面板:1、INPUT:输入插孔,接电吉他2、LEVEL:输出音量(电平)调节旋钮3、OUTPUT R:右声道输出插孔,接音箱等设备4、OUTPUT L(MONO):左声道输出插孔,若非立体声输出,使用此插孔接音箱等设备5、PHONES:耳机插孔,此插孔为3.5mm立体声输出小插孔,也可以利用此插孔接调音台6、SEND:外部效果器(效果设备)输出插孔,接外部效果器(效果设备)的输入插孔7、RETURN:外部效果器(效果设备)输入插孔,接外部效果器(效果设备)的输出插孔8、SUB EXP PEDAL:外部表情踏板接入插孔9、MIDI IN:MIDI输入插孔10、MIDI OUT:MIDI输出插孔11、POWER:GT电源开关按钮12、AC IN 14V:14V直流变压器电源输入插孔=====================================================================二、连接将GT与吉他、音箱等设备连接好后,接通电源。
外文翻译---基于离散混沌映射的图像加密并行算法

3.转换
3.1.A-转换
在A转换中,A代表加,能被形式化的定义如下:
a+b=c(1)
加法被定义为按位与操作
转换A有三个基本性质:
(2.1)a+a=0
(2.2)a+b=b+a(2)
(2.3)(a+b)+c=a+(b+c)
在并行模式计算时,许多的PE可以同时读取或写入相同的内存区域(即临界区),
这往往会导致意想不到的执行程序。因此,有必要在关键区域使用一些并行技术管理。
2.2.并行图像的加密框架
为了满足上述要求,我们提出了一个并行图像加密的框架,这是一个四个步骤的过程:
步骤1:整个图像被划分成若干块。
步骤2:每个PE负责确定数量块。一个区域内的像素可以充分使用有效的混乱和扩散进行操作加密。
附件C:译文
基于离散混沌映射的图像加密并行算法
摘要:
最近,针对图像加密提出了多种基于混沌的算法。然而,它们都无法在并行计算环境中有效工作。在本文中,我们提出了一个并行图像加密的框架。基于此框架内,一个使用离散柯尔莫哥洛夫流映射的新算法被提出。它符合所有并行图像加密算法的要求。此外,它是安全、快速的。这些特性使得它是一个很好的基于并行计算平台上的图像加密选择。
这个框架可以非常有效的实现整个图像的扩散。但是,它是不适合在并行计算环境中运行。这是因为当前像素的处理无法启动直到前一个像素已加密。即使有多个处理元素(PE),这种计算仍然是在一个串行模式下工作。此限制了其应用平台,因为许多基于FPGA / CPLD或者数字电路的设备可以支持并行处理。随着并行计算技术的应用,加密速度可以大大加快。
霍尼韦尔近地告警手册

MK V MK VI MK VII MK VIII MK XXIIEnhanced Ground Proximity Warning SystemsLine Maintenance ManualDocument No: 060-4199-180, Rev FRelease date: 23 Jun 2009Honeywell International, Inc. Redmond, Washington 98073-9701P R O D U C T I O N - R e l e a s e - 25 J u n 2009 15:05:03 M S T - P r i n t e d o n 18 A u g 2009This document is an unpublished work.Copyright 2005, 2009 Honeywell International, Inc.All rights reserved.This document and all information and expression contained herein are theproperty of Honeywell and is provided to the recipient in confidence on a“need to know” basis. Your use of this document is strictly limited to alegitimate business purpose requiring the information contained therein.Your use of this document constitutes acceptance of these terms.Typed signatures constitute approval. Actual Signatures are on file at Honeywell in Redmond, WA.DRAWN R. Halbert 23 FEB 00CHECKENGR R. Halbert 23 FEB 00MFGQAAPVD G. Gilliland 23 FEB 00APVDREVISIONSSHT REV DESCRIPTION DATEAPPROVEDAll Initialrelease.Reason 01 Severity 10 23 FEB 0023 FEB 00R. HalbertG. GillilandAll A Directupdate:Incorporate –008 changesReason 01 Severity 10 07 AUG 0107 AUG 01R. HendersonL. MatterAll B Direct update to incorporate EGPWC/GNSSU part numbers and troubleshooting information. Revised Level 2descriptions.Reason 01 Severity 10 13 FEB 0213 FEB 02B. BreenG. GillilandAll C Direct update to incorporate Airbus and Boeing part number changes, Revised Flight History Download Card P/Ns,reformatted TOC and added the following RAAS-relatedsections:(1) Section 2.2.15(2) “Application Software Version Invalid” and “RCD Failed”(MK V/VII) aural annunciations to Table 3-2.(3) Sections 3.8.42 and 3.8.43(4) Appendix CReason 01 Severity 10 08 JAN 0409 JAN 04J. CastroL. MatterAll D 1) Deleted “Revisions Status of Sheets Index” table onSheet 3.2) Section 3.2 (Troubleshooting Guide): Added thestatement “(this sentence does not apply to internalGPS engines)” after the existing sentence “If no faultsare present, verify that position data from the GPS, IRS,or FMC is correct”.3) Section 6.1.2 (Installation): Added step 6 to account forRAAS equipped EGPWC units.4) Appendix C:- Revised Table B1-1 to C1-1.- Revised Table B1-2 to C1-2.- Table C1-2: Added note that “Operators/Installers mustrefer to their Instructions for Continued Airworthiness(ICA) documentation to identify the approved RCDconfigurations specific to their aircraft type/model havingRAAS installed”.Reason 01 Severity 10 27 SEP 0430 SEP 04K. ChristoffersonS. WrightREVISIONSSHT REV DESCRIPTION DATEAPPROVEDAll E Section 3.3.2 – added RAAS maintenance message to short Level 1 Self-Test sequence and two notes, one to ensureRAAS messages only occur if RAAS is activated and one toexplain the GPS NOT NAVIGATING enunciation.Section 3.5 - added note to ensure RAAS message onlyoccurs if RAAS is activated.Section 6.1.2 – modified step 6 to add Level 3 Self-Test.Reason 01 Severity 10 01 FEB 0503 FEB 05S. WrightK. ChristoffersonAll F Updated per ECO-65820Updated document to include MK XXII helicopter EGPWSand to include software -230-230 changes for RAAS andnewly introduced optional functions for Stabilized ApproachMonitor, Long Landing Monitor, Altimeter Monitor, andTakeoff Flap Configuration Monitor.EFF PT: 10 23 JUN 09 J. MulkinsSee AeroPDMfor additionalapprovalsTABLE OF CONTENTS1INTRODUCTION (8)1.1S COPE (8)1.2A PPLICABILITY (8)1.3R EFERENCE D OCUMENTS (9)2DESCRIPTION AND OPERATION (10)2.1G ENERAL S YSTEM D ESCRIPTION (10)2.1.1Enhanced Ground Proximity Warning Computer (EGPWC) (11)2.2O PERATION (11)2.2.1Mode 1 – Excessive Descent Rate (11)2.2.2Mode 2A/2B - Terrain Closure Rate (11)2.2.3Mode 3 - Descent After Takeoff (11)2.2.4Mode 4A/4B/4C - Unsafe Terrain Clearance (11)2.2.5Mode 5 - Descent Below Glideslope (12)2.2.6Mode 6 - Advisory Callouts (optional) (12)2.2.7Mode 7 - Windshear Detection (Optional for MK V/VII only) (14)2.2.8Envelope Modulation (not available in MK VI/VIII -001) (14)2.2.9Terrain Clearance Floor and Runway Field Clearance Floor (14)2.2.10Terrain Alerting and Display (optional) (15)2.2.11Peaks Display Mode (optional) (16)2.2.12Geometric Altitude (GPS Required) (16)2.2.13Weather Radar AutoTilt (MK V and MK VII only) (16)2.2.14System Display and Annunciation (17)2.2.15Runway Awareness and Advisory System (option for MK V and MK VII only) (17)2.2.16Stabilized Approach Monitor (option for MK V and MK VII only) (17)2.2.17Altimeter Monitor (option for MK V and MK VII only) (17)2.2.18Takeoff Flap Configuration Monitor (option for MK V and MK VII only) (18)2.2.19Long Landing Monitor (option for MK V and MK VII only) (18)2.2.20Lamp Format (19)2.3S YSTEM M AINTENANCE (20)2.3.1Maintenance Philosophy (20)2.3.2System Operation During an Inop Condition (20)2.3.3BIT Description (20)2.3.4EGPWC Front Panel (21)2.3.5Self-Test Functions (22)3FAULT ISOLATION (TROUBLESHOOTING) (23)3.1G ENERAL (23)3.2T ROUBLESHOOTING G UIDE (23)3.3L EVEL 1S ELF-T EST -G O/N O G O T EST (24)3.3.1Self-Test Preamble (24)3.3.2Short Level 1 Self-Test (25)3.3.3Long Level 1 Self-Test (26)3.4L EVEL 2S ELF-T EST -C URRENT F AULTS (26)3.4.1Current Faults - Internal (27)3.4.2Current Faults - External (27)3.5L EVEL 3S ELF-T EST -S YSTEM C ONFIGURATION (33)3.6L EVEL 4S ELF-T EST -F AULT H ISTORY (36)3.7L EVEL 5S ELF-T EST -A LERT H ISTORY (37)3.8L EVEL 6S ELF-T EST -D ISCRETE I NPUT T EST (37)3.8.1ARINC 552 / ALT 55 Radio Altitude Validity Flag Discretes (38)3.8.2GND Landing Gear Discrete (38)3.8.3+28V Landing Gear Discrete (38)3.8.4GND Landing Flap Discrete or Flap Override (39)3.8.5+28V Landing Flap or Flap Override Discrete (39)3.8.6Flap Position Discretes (40)3.8.7Self-Test Discrete (40)3.8.8Steep Approach Discretes (40)3.8.9GND ILS Tuned Discrete (41)3.8.10+28V ILS Tuned Discrete (41)3.8.11Glideslope Validity Discretes (41)3.8.12GND Glideslope Inhibit Discrete (42)3.8.13+28 V Glideslope Inhibit Discrete (42)3.8.14GND Glideslope Cancel Discrete (42)3.8.15Decision Height Discrete (42)3.8.16Mode 6 Volume Control Discrete (43)3.8.17Callouts Enable Discrete (MK V and MK VII only) (43)3.8.18GND Audio Suppress(Inhibit)/All Modes Inhibit Discrete (43)3.8.19+28 V Audio Suppress(Inhibit)/All Modes Inhibit Discrete (43)3.8.20AOA Validity Discretes (MK V and MK VII only) (44)3.8.21Display Select Discretes (44)3.8.22Terrain Awareness & TCF Inhibit (44)3.8.23Simulator Reposition (MK V and MK VII only) (44)3.8.24Weather Radar On/Off (45)3.8.25Localizer Validity Discretes (MK V and MK VII only) (45)3.8.26Attitude Validity Discretes (45)3.8.27Airspeed Validity Discrete (45)3.8.28Barometric Altitude Rate Validity Discretes (46)3.8.29Acceleration Self-Test In Progress Discrete (46)3.8.30Longitudinal Acceleration Validity Discrete (46)3.8.31Normal Acceleration Validity Discrete (46)3.8.32Magnetic Heading Validity Discrete (47)3.8.33AOA Vane Heater Discrete (47)3.8.34PLI Deselect Switch Discretes (MK V and MK VII only) (47)3.8.35Autopilot Disconnect Discretes (47)3.8.36Tactical Select Discrete (48)3.8.37Altitude Alert Discrete (48)3.8.38Corrected Barometric Altitude Validity Discrete (48)3.8.39Momentary Flap Override Discrete (48)3.8.40Weight On Wheels Discrete (49)3.8.41GPWS Inhibit Discrete (49)3.8.42RAAS EnABLE Discrete (MK V and MK VII Only) (49)3.8.43RAAS Inhibit Discrete (MK V and MK VII Only) (49)3.8.44Stabilized Approach Monitor Enable Discrete (MK V and MK VII Only) (50)3.8.45Stabilized approach Monitor Inhibit Discrete (MK V and MK VII Only) (50)4MAINTENANCE PRACTICES (51)4.1G ENERAL (51)4.2D ATABASE U PDATE (51)4.2.1Database Update Frequency (51)4.2.2Loading a Database (51)4.3F LIGHT H ISTORY D OWNLOADING (52)4.3.1Obtaining an EGPWS Flight History Download Card (52)4.3.2Download Procedure (52)4.3.3Transcription of the PCMCIA Card (53)4.4P ROGRAMMING THE C ONFIGURATION M ODULE (MK VI,MK VIII,MK XXII ONLY) (54)4.4.1Configuration Module Reprogramming (MK VI, MK VIII, MK XXII only) (55)5SERVICING (57)5.1G ENERAL (57)6REMOVAL/INSTALLATION (57)6.1EGPWC (57)6.1.1Removal (57)6.1.2Installation (57)6.2C ONFIGURATION M ODULE (MK VI,MK VIII, AND MK XXII ONLY) (57)6.2.1Removal (57)6.2.2Installation (57)6.3D ISPLAY S WITCHING R ELAYS (IF INSTALLED) (58)6.3.1Removal (58)6.3.2Installation (58)7ADJUSTMENT/TEST (58)7.1A DJUSTMENT (58)7.2T EST (58)7.2.1EGPWS Ground Tests (58)8INSPECTION/CHECK (59)8.1G ENERAL (59)9CLEANING/PAINTING (59)9.1G ENERAL (59)10REPAIRS (59)10.1G ENERAL (59)11APPENDIX A: WINVIEWS (60)12APPENDIX B: TROUBLESHOOTING DO’S AND DO NOT’S (61)13APPENDIX C: RAAS MAINTENANCE MESSAGES (AURAL & DISPLAYED) (62)14APPENDIX D: STABILIZED APPROACH MONITOR MAINTENANCE MESSAGES (64)15APPENDIX E: ALTIMETER MONITOR MAINTENANCE MESSAGES (65)16APPENDIX F: TAKEOFF FLAP CONFIGURATION MONITOR MAINTENANCE MESSAGES (66)17APPENDIX G: LONG LANDING MONITOR MAINTENANCE MESSAGES (67)1 INTRODUCTION1.1 SCOPEThis document provides information about the Enhanced Ground Proximity Warning System (EGPWS) with respect to Line Maintenance Operations. This includes Description and Operation, Troubleshooting, Removal and Installation, Adjustment and Test, and other related information. It is intended that the information in this document be combined with detailed aircraft installation documentation for operator specific line maintenance procedures. 1.2 APPLICABILITYThis manual is applicable to the MK V, MK VI, MK VII, MK VIII, and MK XXII EGPWS with the following part numbers and general description:MK V EGPWC PART NUMBERS MK VII EGPWC PART NUMBERS 965-0976-003-XXX-XXX 115 VAC, -40° to +70°965-1076-001-XXX-XXX 115 VAC, -40° to +70°965-0976-020-XXX-XXX 115 VAC, Internal 8 channelGPS, -40° to +70°965-1076-020-XXX-XXX115 VAC, Internal 8 channelGPS, -40° to +70°965-0976-040-XXX-XXX 28 VDC, -55° to +70°965-1076-030-XXX-XXX 28 VDC, Internal 8 channel GPS, 55° to +70°965-0976-060-XXX-XXX 115 VAC, Integral GNSSU,-40° to +70°965-1076-040-XXX-XXX 28 VDC, -55° to +70°965-1676-XXX (Airbus P/N) 115 VAC, -40° to +70°965-1076-060-XXX-XXX 115 VAC, Integral GNSSU, -40° to +70°965-1690-XXX (Boeing P/N) 115 VAC, -40° to +70°MK VI EGPWC PART NUMBERS MK VIII EGPWC PART NUMBERS MK XXII EGPWC PART NUMBERS965-1176-XXX 28 VDC, -55° to +70°965-1206-XXX 28 VDC, -55° to +70°965-1590-XXX 28 VDC, Internal 8 channel GPS, -55° to +70°965-1186-XXX 28 VDC, Internal 8 channelGPS, -55° to +70°965-1216-XXX28 VDC, Internal 8 channelGPS, -55° to +70°965-1595-XXX28 VDC, Improved CPU,Internal 8 channel GPS,-55° to +70°965-1180-XXX 28 VDC, Improved CPU,-55° to +70°965-1210-XXX28 VDC, Improved CPU,-55° to +70°965-1190-XXX 28 VDC, Improved CPU,Internal 8 channel GPS,-55° to +70°965-1220-XXX28 VDC, Improved CPU,Internal 8 channel GPS,-55° to +70°TABLE 1-1 EGPWC Part NumbersNOTE: X’s represent variable values defining a specific application and configuration software version (i.e., -230-230 for MK V/VII or -011 for MK VI/VIII/XXII original model or -026 for MK VI/VIII/XXII with improved CPU).MK V & MK VII EGPWC (left) and MK VI, MK VIII, MK XXII EGPWC (right)Figure 1-1 - Enhanced Ground Proximity Warning Computers1.3 REFERENCE DOCUMENTSThe following documents are identified as additional EGPWS references:MK V and MK VII:965-0976-603.........Product Specification for the EGPWS (MK V and MK VII)060-4404-000………Product Description for Runway Awareness and Advisory System (RAAS) prior to -230-230 060-4564-000………Product Description, Flight Safety Functions of the EGPWS993-0976-401.........Interface Control Document for the Mark V EGPWS993-1076-401.........Interface Control Document for the Mark VII EGPWS060-4199-125.........Installation Design Guide for the MK V EGPWS060-4199-225.........Installation Design Guide for the MK VII EGPWS060-4241-000.........MK V and MK VII EGPWS Pilot Guide060-4267-000.........EGPWS Terrain Database Airport Coverage List (MK V and MK VII)060-4353-000.........EGPWS Terrain Database Obstacle Coverage ChartMK VI, MK VIII, MK XXII:965-1176-601.........Product Specification for the MK VI and MK VIII EGPWS (original model)965-1180-601.........Product Specification for the MK VI and MK VIII EGPWS (with improved CPU)965-1590-601.........Product Specification for the MK XXII EGPWS (original model)965-1595-601.........Product Specification for the MK XXII EGPWS (with improved CPU)993-1176-401.........Interface Control Document for the Mark VI/VIII/XXII EGPWS (original model)993-1180-401.........Interface Control Document for the Mark VI/VIII/XXII EGPWS (with improved CPU)060-4314-125.........Installation Design Guide for the MK VI/VIII EGPWS (original model)060-4314-150………Installation Design Guide for the MK VI/VIII EGPWS (with improved CPU)060-4314-225.........Installation Design Guide for the MK XXII EGPWS (original model)060-4314-250………Installation Design Guide for the MK XXII EGPWS (with improved CPU)060-4314-000.........MK VI and MK VIII EGPWS Pilot Guide060-4314-200.........MK XXII EGPWS Pilot Guide060-4326-000.........EGPWS Terrain Database Airport Coverage List (MK VI and MK VIII)2 DESCRIPTION AND OPERATION2.1 GENERAL SYSTEM DESCRIPTIONThe purpose for the Enhanced Ground Proximity Warning System (EGPWS) is to help prevent accidents caused by Controlled Flight Into Terrain (CFIT), obstacles, or severe windshear. The Enhanced Ground Proximity Warning Computer (EGPWC) accepts a variety of aircraft sensors and system inputs and applies alerting algorithms to provide the flight crew with aural messages and visual annunciations when the boundaries of alerting envelopes are exceeded. A graphic depiction of terrain and obstacles within the range selected on an EFIS or Weather Radar display may be configured for enhanced situational awareness with respect to terrain and obstacles. Figure 2-1 provides an overall system block diagram.Figure 2-1: Enhanced Ground Proximity Warning SystemThe EGPWS is comprised of the following:•Aircraft sensors and systems providing input signals•The Enhanced Ground Proximity Warning Computer (EGPWC)•Flight deck audio systems (speaker and interphone)•Alert lamps and/or EFIS or EICAS displays (for alert and system status messages)•EFIS Navigation Display (ND), Multi-Function Display (MFD), or Weather Radar Indicator for display of terrain •Switching relay(s) when required for switching display inputs from weather display to terrain display•GPS antenna for direct connection to EGPWC with internal GPS sensors2.1.1 ENHANCED GROUND PROXIMITY WARNING COMPUTER (EGPWC)All EGPWS functions are processed by a single Line Replaceable Unit (LRU) called the Enhanced Ground Proximity Warning Computer (EGPWC).The MK V and MK VII EGPWC are digitally controlled computers housed in a 2 MCU ARINC 600-6 form factor chassis intended for Air Transport type aircraft. Installation configuration is defined by program pin strapping in the aircraft.The MK VI and MK VIII EGPWC are digitally controlled computers housed in a non-ARINC form factor chassis intended for Business and General Aviation and Regional Turboprop type aircraft. These models have fewer interface and functional options. The installation configuration is defined in a programmed Configuration Module installed in the aircraft.The MK XXII EGPWC is a digitally controlled computer housed in a non-ARINC form factor chassis intended for various rotorcraft. Similar to the MK VI and MK VIII EGPWC, this model has fewer interface and functional options. The installation configuration is defined in a programmed Configuration Module installed in the helicopter.The EGPWC receives information in AC, DC, discrete, and synchro analog formats, and RS-232, RS-422, ARINC 429 or ARINC 575 digital formats. Discrete signals can be either ground or +28V discretes. The EGPWC provides discrete, audio and ARINC 429 outputs for alerts and system status, and video (ARINC 453/708) for terrain display. The EGPWC is rack mounted and does not require any forced air cooling when operated within the normal operating temperature range given in the Table 1-1.2.2 OPERATION2.2.1 MODE 1 – EXCESSIVE DESCENT RATEMode 1 provides audio and visual alerts for excessive descent rates into terrain. When the EGPWS caution alert envelope is penetrated, the message “SINKRATE” is enunciated and EGPWS alert lights illuminate. Continuing the excessive descent rate into the EGPWS warning alert envelope results in a “PULL-UP” enunciation and EGPWS alert lights illuminated. Mode 1 is desensitized to eliminate unwanted (nuisance) alerts when the EGPWS determines that the aircraft is above a Glideslope beam. In some fixed-wing applications, Mode 1 is also desensitized when Steep Approach or Flap Override is active. In helicopter applications, Mode 1 is disabled when autorotation is detected.2.2.2 MODE 2A/2B - TERRAIN CLOSURE RATEMode 2 provides audio and visual alerts for dangerously high terrain closure rates. Two sub-modes, referred to as Mode 2A and 2B, are defined. Mode 2A is active when flaps are not in the landing position and the aircraft is not on an ILS approach within ± 2 dots of glideslope center. Mode 2B is active when the flaps are in the landing position or while on an ILS approach within ± 2 dots of glideslope deviation. When the caution alert envelope is penetrated, the message “TERRAIN, TERRAIN” is enunciated and EGPWS alert lights illuminate. Continuing the high terrain closure rate into the warning alert envelope results in a “PULL-UP” enunciation and EGPWS alert lights illuminated.2.2.3 MODE 3 - DESCENT AFTER TAKEOFFMode 3 provides audio and visual alerts for excessive altitude loss after takeoff, or after a go-around from below 245 feet above ground level (AGL), when flaps and gear are not in the landing configuration. Penetrating the Mode 3 alert envelope causes the voice message “DON’T SINK, DON’T SINK” and illumination of EGPWS alert lights.2.2.4 MODE 4A/4B/4C - UNSAFE TERRAIN CLEARANCEMode 4 provides audio and visual alerts for unsafe terrain clearance with respect to phase of flight, height above ground, and speed. Three sub-modes, referred to as Mode 4A, 4B, and 4C, are defined. Mode 4A is active during cruise and approach with landing gear up. Mode 4B is active during cruise and approach with landing gear down and flaps up. Mode 4C is active during takeoff when either gear or flaps are not in the landing configuration. The aural enunciations for Mode 4A are “TOO LOW TERRAIN” or “TOO LOW GEAR” depending on airspeed. Mode 4Bprovides “TOO LOW TERRAIN” or “TOO LOW FLAPS” depending on airspeed. Mode 4C provides “TOO LOW TERRAIN”. EGPWS alert lights are illuminated during these alerts.2.2.5 MODE 5 - DESCENT BELOW GLIDESLOPEMode 5 provides audio and visual alerts for excessive glideslope deviation when the aircraft descends below the glideslope beam on front-course ILS approaches. Two levels of alerting are provided. If the aircraft is below 1000 feet AGL and gets to or exceeds 1.3 dots glideslope deviation (fly-up), a ‘soft’ (reduced volume) “GLIDESLOPE” is enunciated. Exceeding 2 dots below 300 feet AGL provides a hard (full volume) “GLIDESLOPE” enunciation. EGPWS alert lights are illuminated during these alerts.2.2.6 MODE 6 - ADVISORY CALLOUTS (OPTIONAL)The EGPWC can be programmed to enunciate Mode 6 Advisory Callouts based on menu selectable options. Mode 6 includes Altitude Awareness, Minimums/Approaching Minimums, and Bank Angle type callouts as defined foreach EGPWS model (refer to an applicable Interface Control Document or Installation Design Guide). The menu selected Advisory Callouts are defined and enabled in the installation configuration. If Altitude Callouts are not enabled, only (DH based) “MINIMUMS” callouts will be provided. The MK XXII offers a tail strike advisory for helicopters.Only aural callouts are provided for Mode 6. EGPWS alert lights are NOT illuminated for Mode 6 callouts. The following table identifies all of the Mode 6 Callouts that are available and the applicability to each model.TABLE 2-1: MODE 6 CALLOUTSMODEL CALLOUT DESCRIPTIONV VI VII VIII XXII “DECISION HEIGHT“ At descent below minimums setting (DH) •# •# # “CHECK HEIGHT” At descent below minimums setting (DH) # # # # •“ALTITUDE-ALTITUDE” At descent below minimums setting (DH) with gear up # # # # •“MINIMUMS” At descent below minimums setting (DH) •# •# # “MINIMUM” At descent below minimums setting (DH) •# •# # “MINIMUMS-MINIMUMS” Atdescent below minimums setting (DH) •••••“DECIDE” At descent below minimums setting (DH) •# •# #“APPROACHING DECISION HEIGHT” At descent below minimums (DH altitude) setting plus100 feet•# •# #“APPROACHING MINIMUMS” At descent below minimums setting (DH altitude) plus80 feet•# •# #“PLUS HUNDRED” At descent below minimums setting (DH altitude) plus100 feet•# •# #“FIFTY ABOVE” At descent below minimums setting (DH altitude) plus50 feet•# •# # “RADIO ALTIMETER” At descent below 2500 feet •# •# # “TWENTY FIVE HUNDRED” At descent below 2500 feet •# •# # “ONE THOUSAND” At descent below 1000 feet ••••# “FIVE HUNDRED” At descent below 500 feet ••••#MODEL CALLOUT DESCRIPTIONV VI VII VIII XXII500 TONE Provides 2 second 960 Hz tone at descent below 500•# •# #feetSMART “FIVE HUNDRED” At descent below 500 feet (only) during non-precision•••••approach“FIVE HUNDRED” Above field callout within 5 nm of a runway in the# •# •#database“FIVE HUNDRED ABOVE” Above field callout within 5 nm of a runway in the# •# •#database“FOUR HUNDRED” At descent below 400 feet ••••# “THREE HUNDRED” At descent below 300 feet ••••# “TWO HUNDRED” At descent below 200 feet •••••“ONE HUNDRED SIXTY” At descent below 160 feet # # # # •“ONE HUNDRED FIFTY” At descent below 150 feet # # # # •“ONE HUNDRED FORTY” At descent below 140 feet # # # # •“ONE HUNDRED THIRTY” At descent below 130 feet # # # # •“ONE HUNDRED TWENTY” At descent below 120 feet # # # # •“ONE HUNDRED TEN” At descent below 110 feet # # # # •“ONE HUNDRED” At descent below 100 feet •••••100 TONE Provides 2 second 700 Hz tone at descent below 100•# •# #feet“EIGHTY” At descent below 80 feet •# •# •“SIXTY” At descent below 60 feet •# •# •“FIFTY” At descent below 50 feet •••••“FOURTY” At descent below 40 feet •••••“THIRTY FIVE” At descent below 35 feet •# •# #35 TONE Provides 1 second 1400 Hz tone at descent below 35•# •# #feet“THIRTY” At descent below 30 feet •••••“TWENTY” At descent below 20 feet •••••20 TONE Provides 1/2 second 2800 Hz tone at descent below•# •# #20 feet“FIFTEEN” At descent below 15 feet •# •# # “TEN“ At descent below 10 feet •••••“FIVE” At descent below 5 feet •# •# # # Not currently identified in any menu option for the model indicated.2.2.6.1 EXCESSIVE BANK ANGLE CALLOUTThe Bank Angle Callout feature provides callout enunciation for excessive bank angles based on altitude and bank angle limits defined by aircraft type. It is intended to enhance situational awareness during intentional or unintentional maneuvering, and for protection against wing or engine strikes when close to the runway.When the bank angle limit is reached, the aural callout “BANK ANGLE, BANK ANGLE” is given. Follow-on aural messages are only provided when the aircraft roll angle increases an additional 20% from the previous callout. Bank Angle Callouts are enabled by the installation configuration.2.2.6.2 TAIL STRIKE CALLOUTA tail strike alert function is provided by the MK XXII for applicable rotary wing aircraft based upon Radio Altitude, Pitch Attitude, Pitch Rate and Barometric Altitude Rate. The voice message “Tail Too Low “ is provided continuously while within the alert boundary. Unique alert boundaries are provided for applicable aircraft types.2.2.7 MODE 7 - WINDSHEAR DETECTION (OPTIONAL FOR MK V/VII ONLY)Mode 7 provides the flight crew with visual and aural alerts for windshear of sufficient magnitude to be potentially hazardous to the aircraft. The system is capable of detecting severe decreasing performance shears (i.e. increasing tailwind/decreasing headwind and/or downdraft) which present an immediate danger to the aircraft. The system is also capable of detecting severe increasing performance shears (increasing headwind/decreasing tailwind and/or up draft). While these shears may not present an immediate danger to the aircraft, these shears can indicate that the atmospheric instability is such that an encounter with a severe decreasing performance shear is likely.A detected increasing performance shear will result in an aural “CAUTION WINDSHEAR” enunciation and cockpit light annunciation when enabled. A detected decreasing performance shear will result in an aural siren followed by “WINDSHEAR, WINDSHEAR, WINDSHEAR” with a corresponding cockpit warning light annunciation.2.2.8 ENVELOPE MODULATION (NOT AVAILABLE IN MK VI/VIII -001)Envelope Modulation provides improved alert protection and expanded alerting margins at identified key locations throughout the world. Due to terrain features at or near certain specific airports, normal operations have resulted in nuisance or missed alerts at these locations in the past. With the introduction of accurate position information and a terrain and airport database, it is now possible to identify these areas and adjust the normal alerting process to compensate for the condition.Modes 4, 5, and 6 are expanded at certain locations to provide alerting protection consistent with normal approaches. Modes 1, 2, and 4 are desensitized at other locations to prevent nuisance alerts that result from unusual terrain or approach procedures. In all cases, very specific information is used to correlate the aircraft position and phase of flight prior to modulating the envelopes. This function is automatic and transparent to crew operation.2.2.9 TERRAIN CLEARANCE FLOOR AND RUNWAY FIELD CLEARANCE FLOORThe Terrain Clearance Floor (TCF) alerting function adds an additional element of protection to the standard Ground Proximity Warning System for fixed-wing aircraft. It creates an increasing terrain clearance envelope around the airport runway to provide CFIT protection against situations where Mode 4 provides limited or no protection. TCF alerts are based on current aircraft location, destination runway center point position, and Radio Altitude (altitude AGL). TCF is active during takeoff, cruise, and final approach. TCF complements the existing Mode 4 protection by providing an alert based on insufficient terrain clearance even when in landing configuration.The TCF function is enhanced in all fixed-wing models (beginning with release –210-210 for the MK V/VII) with the addition of a Runway Field Clearance Floor (RFCF) alerting function. RFCF is based on current aircraft location, destination runway center point position, and Geometric Altitude or altitude Above Sea Level (ASL) relative to the destination runway. RFCF provides short landing protection for runways that are significantly higher than the surrounding terrain.。
Measurements of Cross Sections and Forward-Backward Asymmetries at the Z Resonance and Dete

a rXiv:h ep-e x /246v116Feb2EUROPEAN ORGANIZATION FOR NUCLEAR RESEARCH CERN-EP/2000-022February 04,2000Measurements of Cross Sections and Forward-Backward Asymmetries at the Z Resonance and Determination of Electroweak Parameters The L3Collaboration Abstract We report on measurements of hadronic and leptonic cross sections and leptonic forward-backward asymmetries performed with the L3detector in the years 1993−95.A total luminosity of 103pb −1was collected at centre-of-mass energies √s ≈m Z ±1.8GeV which corresponds to 2.5million hadronic and 245thousand leptonic events selected.These data lead to a significantly improved determination of Z parameters.From the total cross sections,combined with our measurements in 1990−92,we obtain the final results:m Z =91189.8±3.1MeV ,ΓZ =2502.4±4.2MeV ,Γhad =1751.1±3.8MeV ,Γℓ=84.14±0.17MeV .An invisible width of Γinv =499.1±2.9MeV is derived which in the Standard Model yields for the number of light neutrino species N ν=2.978±0.014.Adding our results on the leptonic forward-backward asymmetries and the tau polarisation,the effective vector and axial-vector coupling constants of the neutral weak current to charged leptons are determined to be ¯g ℓV =−0.0397±0.0017and ¯g ℓA =−0.50153±0.00053.Includingour measurements of the Z →b ¯b forward-backward and quark charge asymmetries a value for theeffective electroweak mixing angle of sin 21IntroductionThe Standard Model(SM)of electroweak interactions[1,2]is tested with great precision by the experiments performed at the LEP and SLC e+e−colliders running at centre-of-mass energies,√on the treatment of the t-channel contributions in e+e−→e+e−(γ)and on technicalities of the fit procedures,respectively.2The L3DetectorThe L3detector[13]consists of a silicon microvertex detector[14],a central tracking chamber, a high resolution electromagnetic calorimeter composed of BGO crystals,a lead-scintillator ring calorimeter at low polar angles[15],a scintillation counter system,a uranium hadron calorime-ter with proportional wire chamber readout and an accurate muon spectrometer.Forward-backward muon chambers,completed for the1995data taking,extend the polar angle coverage of the muon system down to24degrees[16]with respect to the beam line.All detectors are installed in a12m diameter magnet which provides a solenoidalfield of0.5T in the central region and a toroidalfield of1.2T in the forward-backward region.The luminosity is measured using BGO calorimeters preceded by silicon trackers[10]situated on each side of the detector.In the L3coordinate system the direction of the e−beam defines the z direction.The xy, or rφplane,is the bending plane of the magneticfield,with the x direction pointing to the centre of the LEP ring.The coordinatesφandθdenote the azimuthal and polar angles.3Data AnalysisThe data collected between1993and1995are split into nine samples according to the year√and the centre-of-mass energy.Data samples atshowers are simulated with the GHEISHA[28]program.The performance of the detector, including inefficiencies and their time dependence as observed during data taking,is taken into account in the simulation.With this procedure,experimental systematic errors on cross sections and forward-backward asymmetries are minimized.4LEP Energy CalibrationThe average centre-of-mass energy of the colliding particles at the L3interaction point is calcu-lated using the results provided by the Working Group on LEP Energy[9].Every15minutes the average centre-of-mass energy is determined from measured LEP machine parameters,ap-plying the energy model which is based on calibration by resonant depolarisation[29].This model traces the time variation of the centre-of-mass energy of typically1MeV per hour.The average centre-of-mass energies are calculated for each data sample individually as luminosity weighted averages.Slightly different values are obtained for different reactions because of small differences in the usable luminosity.The errors on the centre-of-mass energies and their correlations for the1994data and for the two scans performed in1993and1995are given in form of a7×7covariance matrix in Table1.The uncertainties on the centre-of-mass energy for the data samples not included in this matrix,i.e.the1993and1995pre-scans,are18MeV and10MeV,respectively.Details of the treatment of these errors in thefits can be found in Appendix B.The energy distribution of the particles circulating in an e+e−-storage ring has afinite width due to synchrotron oscillations.An experimentally observed cross section is therefore a convolution of cross sections at energies which are distributed around the average value in a gaussian form.The spread of the centre-of-mass energy for the L3interaction point as obtained from the observed longitudinal length of the particle bunches in LEP is listed in Table2[9]. The time variation of the average energy causes a similar,but smaller,effect which is included in these numbers.All cross sections and forward-backward asymmetries quoted below are corrected for the energy spread to the average value of the centre-of-mass energy.The relative corrections on the measured hadronic cross sections amount to+1.7per mill(‰)at the Z pole and to−1.1‰and−0.6‰at the peak−2and peak+2energy,respectively.The absolute corrections on the forward-backward asymmetries are very small.The largest correction is−0.0002for the muon and tau peak−2data sets.The error on the energy spread is propagated into thefits,resulting in very small contributions to the errors of thefitted parameters(see Appendix B).The largest effect is on the total width of the Z,contributing approximately0.3MeV to its error.During the operation of LEP,no evidence for an average longitudinal polarisation of the electrons or positrons has been observed.Stringent limits on residual polarisation during lumi-nosity runs are set such that the uncertainties on the determination of electroweak observables are negligible compared to their experimental errors[30].The determination of the LEP centre-of-mass energy in1990−92is described in Refer-ences[31].From these results the LEP energy error matrix given in Table3is derived.5Luminosity MeasurementThe integrated luminosity L is determined by measuring the number of small-angle Bhabha interactions e+e−→e+e−(γ).For this purpose two cylindrical calorimeters consisting of arraysof BGO crystals are located on either side of the interaction point.Both detectors are dividedinto two half-rings in the vertical plane to allow the opening of the detectors duringfilling ofLEP.A silicon strip detector,consisting of two layers measuring the polar angle,θ,and one layer measuring the azimuthal angle,φ,is situated in front of each calorimeter to preciselydefine thefiducial volume.A detailed description of the luminosity monitor and the luminosity determination can be found in Reference[10].The selection of small-angle Bhabha events is based on the energy depositions in adjacentcrystals of the BGO calorimeters which are grouped to form clusters.The highest-energy cluster on each side is considered for the luminosity analysis.For about98%of the cases a hitin the silicon detectors is matched with a cluster and its coordinate is used;otherwise the BGOcoordinate is retained.The event selection criteria are:1.The energy of the most energetic cluster is required to exceed0.8E b and the energy onthe opposite side must be greater than0.4E b,where E b is the beam energy.If the energyof the most energetic cluster is within±5%of E b the minimum energy requirement onthe opposite side is reduced to0.2E b in order to recover events with energy lost in the gaps between crystals.The distributions of the energy of the most energetic cluster andthe cluster on the opposite side as measured in the luminosity monitors are shown in Figure1for the1993data.All selection cuts except the one under study are applied.2.The cluster on one side must be confined to a tightfiducial volume:•32mrad<θ<54mrad;|φ−90◦|>11.25◦and|φ−270◦|>11.25◦.The requirements on the azimuthal angle remove the regions where the half-rings of thedetector meet.The cluster on the opposite side is required to be within a largerfiducialvolume:•27mrad<π−θ<65mrad;|φ−90◦|>3.75◦and|φ−270◦|>3.75◦.This ensures that the event is fully contained in the detectors and edge effects in the reconstruction are avoided.3.The coplanarity angle∆φ=φ(z<0)−φ(z>0)between the two clusters must satisfy|∆φ−180◦|<10◦.The distribution of the coplanarity angle is shown in Figure2.Very good agreement with theMonte Carlo simulation is observed.Four samples of Bhabha events are defined by applying the tightfiducial volume cut to oneof theθ-measuring silicon layers.Taking the average of the luminosities obtained from thesesamples minimizes the effects of relative offsets between the interaction point and the detectors. The energy and coplanarity cuts reduce the background from random beam-gas coincidences.The remaining contamination is very small:(3.4±2.2)·10−5.This number is estimated using the sidebands of the coplanarity distribution,10◦<|∆φ−180◦|<30◦,after requiring that neither of the two clusters have an energy within±5%of E b.The accepted cross section is determined from Monte Carlo e+e−→e+e−(γ)samples gen-√erated with the BHLUMI event generator at afixed centre-of-mass energy ofs=91.25GeV the acceptedcross section is determined to be69.62nb.The statistical error on the Monte Carlo sample con-tributes0.35‰to the uncertainty of the luminosity measurement.The theoretical uncertainty on the Bhabha cross section in ourfiducial volume is estimated to be0.61‰[12].The experimental errors of the luminosity measurement are small.Important sources of systematic errors are:geometrical uncertainties due to the internal alignment of the silicon detectors(0.15‰to0.27‰),temperature expansion effects(0.14‰)and the knowledge on the longitudinal position of the silicon detectors(0.16‰to0.60‰).The precision depends on the accuracy of the detector surveys and on the stability of the detector and wafer positions during the different years.The polar angle distribution of Bhabha scattering events used for the luminosity measure-ment is shown in Figure3.The structure seen in the central part of the+z side is due to the flare in the beam pipe on this side.The imperfect description in the Monte Carlo does not pose any problem as it is far away from the edges of thefiducial volume.The overall agreement between the data and Monte Carlo distributions of the selection quantities is good.Small discrepancies in the energy distributions at high energies are due to contamination of Bhabha events with beam-gas interactions and,at low energies,due to an imperfect description of the cracks between crystals.The selection uncertainty is estimated by varying the selection criteria over reasonable ranges and summing in quadrature the resulting contributions.This procedure yields errors between0.42‰and0.48‰for different years.The luminosities determined from the four samples described above agree within these errors.The trigger inefficiency is measured using a sample of events triggered by only requiring an energy deposit exceeding30GeV on one side.It is found to be negligible.The various sources of uncertainties are summarized in bining them in quadra-ture yields total experimental errors on the luminosity of0.86‰,0.64‰and0.68‰in1993,1994 and1995.Correlations of the total experimental systematic errors between different years are studied and the correlation matrix is given in Table5.The error from the theory is fully correlated.Because of the1/s dependence of the small angle Bhabha cross section,the uncertainty on the centre-of-mass energies causes a small additional uncertainty on the luminosity measure-ment.For instance,this amounts to0.1‰for the high statistics data sample of1994.This effect is included in thefits performed in Section12and13,see Appendix B.The statistical error on the luminosity measurement from the number of observed small angle Bhabha events is also included in thosefits.Table6lists the number of observed Bhabha events for the nine data samples and the corresponding errors on cross section measurements.√Combining all data sets taken in1993−95at6e+e−→hadrons(γ)Event SelectionHadronic Z decays are identified by their large energy deposition and high multiplicity in theelectromagnetic and hadron calorimeters.The selection criteria are similar to those applied in our previous analysis[4]:1.The total energy observed in the detector,E vis,normalised to the centre-of-mass energy√must satisfy0.5<E vis/√s′is the effective centre-of-mass energy after initial state s′>0.1√s is estimated to be photon radiation.The acceptance for events in the data withnegligible.They are not considered as part of the signal and hence not corrected for.The interference between initial andfinal state photon radiation is not accounted for in the event generator.This effect modifies the angular distribution of the events in particular at very low polar angles where the detector inefficiencies are largest.However,the error from the imperfect simulation on the measured cross section,which includes initial-final state interference as part of the signal,is estimated to be very small(≪0.1pb)in the centre-of-mass energyrange considered here.Quark pairs originating from pair production from initial state radiation√are considered as part of the signal if their invariant mass exceeds50%ofDifferences of the implementation of QED effects in both programs are studied and found tohave negligible impact on the acceptance.Hadronic Z decays are triggered by the energy,central track,muon or scintillation counter multiplicity triggers.The combined trigger efficiency is obtained from the fraction of events with one of these triggers missing as a function of the polar angle of the event thrust axis. This takes into account most of the correlations among triggers.A sizeable inefficiency is only observed for events in the very forward region of the detector,where hadrons can escape through the beam pipe.Trigger efficiencies,including all steps of the trigger system,between99.829% and99.918%are obtained for the various data sets.Trigger inefficiencies determined for data sets taken in the same year are statistically bining those data sets results in statistical errors of at most0.12‰which is assigned as systematic error to all data sets.The background from other Z decays is found to be small:2.9‰essentially only from e+e−→τ+τ−(γ).The uncertainty on this number is negligible compared to the total systematic error.The determination of the non-resonant background,mainly e+e−→e+e−hadrons,is based on the measured distribution of the visible energy shown in Figure5.The Monte Carlo program PHOJET is used to simulate two-photon collision processes.The absolute cross section isderived by scaling the Monte Carlo to obtain the best agreement with our data in the low end√of the E vis spectrum:0.32≤E vis/s is observed.This is in agreement with results of a similar calculation performed with the DIAG36program.Beam related background(beam-gas and beam-wall interactions)is small.To the extent that the E vis spectrum is similar to that of e+e−→e+e−hadrons,it is accounted for by determining the absolute normalisation from the data.As a check,the non-resonant background is estimated by extrapolating an exponential dependence of the E vis spectrum from the low energy part into the signal region.This method yields consistent results.Based on these studies we assign an error on the measured hadron cross section of3pb due to the understanding of the non-resonant background.This errorassignment is supported by our measurements of the hadronic cross section at high energies √(130GeV≤certainties which scale with the cross section and absolute uncertainties are separated because they translate in a different way into errors on Z parameters,in particular on the total width. The scale error is further split into a part uncorrelated among the data samples,in this case consisting of the contribution of Monte Carlo statistics,and the rest which is taken to be fully correlated and amounts to0.39‰.The results of the e+e−→hadrons(γ)cross section measurements are discussed in Sec-tion10.7e+e−→µ+µ−(γ)Event SelectionThe selection of e+e−→µ+µ−(γ)in the1993and1994data is similar to the selection applied in previous years described in Reference[4].Two muons in the polar angular region|cosθ|<0.8 are required.Most of the muons,88%,are identified by a reconstructed track in the muon spectrometer.Muons are also identified by their minimum ionising particle(MIP)signature in the inner sub-detectors,if less than two muon chamber layers are hit.A muon candidate is denoted as a MIP,if at least one of the following conditions is fulfilled:1.A track in the central tracking chamber must point within5◦in azimuth to a cluster inthe electromagnetic calorimeter with an energy less than2GeV.2.On a road from the vertex through the barrel hadron calorimeter,at leastfive out of amaximum of32cells must be hit,with an average energy of less than0.4GeV per cell.3.A track in the central chamber or a low energy electromagnetic cluster must point within10◦in azimuth to a muon chamber hit.In addition,both the electromagnetic and the hadronic energy in a cone of12◦half-opening angle around the MIP candidate,corrected for the energy loss of the particle,must be less than 5GeV.Events of the reaction e+e−→µ+µ−(γ)are selected by the following criteria:1.The event must have a low multiplicity in the calorimeters N cl≤15.2.If at least one muon is reconstructed in the muon chambers,the maximum muon momen-tum must satisfy p max>0.6E b.If both muons are identified by their MIP signature there must be two tracks in the central tracking chamber with at least one with a transverse momentum larger than3GeV.3.The acollinearity angleξmust be less than90◦,40◦or5◦if two,one or no muons arereconstructed in the muon chambers.4.The event must be consistent with an origin of an e+e−-interaction requiring at least onetime measurement of a scintillation counter,associated to a muon candidate,to coincide within±3ns with the beam crossing.Also,there must be a track in the central tracking chamber with a distance of closest approach to the beam axis of less than5mm.As an example,Figure11shows the distribution of the maximum measured muon momen-tum for candidates in the1993−94data compared to the expectation for signal and backgroundprocesses.The acollinearity angle distribution of the selected muon pairs is shown in Figure12. The experimental angular resolution and radiation effects are well reproduced by the Monte Carlo simulation.The analysis of the1995data in addition uses the newly installed forward-backward muon chambers.Thefiducial volume is extended to|cosθ|<0.9.Each event must have at least one track in the central tracking chamber with a distance of closest approach in the transverse plane of less than1mm and a scintillation counter time coinciding within±5ns with the beam crossing.The rejection of cosmic ray muons in the1995data is illustrated in Figure13.For events with muons reconstructed in the muon chambers the maximum muon momentum must be larger than2µ+µ−(γ)are summarised in Table8.Resonant four-fermionfinal states with a high-mass muon pair and a low-mass fermion pair are accepted.These events are considered as part of the signal if the invariant mass of√the muon pair exceeds0.5,(2)σF+σBwhereσF is the cross section for events with the fermion scattered into the hemisphere which is forward with respect to the e−beam direction.The cross section in the backward hemisphere is denoted byσB.Events with hard photon bremsstrahlung are removed from the sample by requiring that the acollinearity angle of the event be less than15◦.The differential cross section in the angular region|cosθ|<0.9can then be approximated by the lowest order angular dependence to sufficient precision:dσ8 1+cos2θ +A FB cosθ,(3) withθbeing the polar angle of thefinal state fermion with respect to the e−beam direction.For each data set the forward-backward asymmetry is determined from a maximum likeli-hoodfit to our data where the likelihood function is defined as the product over the selected events labelled i of the differential cross section evaluated at their respective scattering angle θi:L= i 3strongly depends on the number of muon chamber layers used in the reconstruction.The charge confusion is determined for each event class individually.The average charge confusion probability,almost entirely caused by muons only measured in the central tracking chamber, is(3.2±0.3)‰,(0.8±0.1)‰and(1.0±0.3)‰for the years1993,1994and1995,respectively, where the errors are statistical.The improvement in the charge determination for1994and 1995reflects the use of the silicon microvertex detector.The correction for charge confusion is proportional to the forward-backward asymmetry and it is less than0.001for all data sets.To estimate a possible bias from a preferred orientation of events with the two muons measured to have the same charge we determine the forward-backward asymmetry of these events using the track with a measured momentum closer to the beam energy.The asymmetry of this subsample is statistically consistent with the standard measurement.Including these like-sign events in the1994sample would change the measured asymmetry by0.0008.Half of this number is taken as an estimate of a possible bias of the asymmetry measurement from charge confusion in the1993−94data.The same procedure is applied to the1995data and the statistical precision limits a possible bias to0.0010.Differences of the momentum reconstruction in forward and backward events would cause a bias of the asymmetry measurement because of the requirement on the maximum measured muon momentum.We determine the loss of efficiency due to this cut separately for forward and backward events by selecting muon pairs without cuts on the reconstructed momentum.No significant difference is observed and the statistical error of this comparison limits the possible effect on the forward-backward asymmetry to be less than0.0004and0.0009for the1993−94 and1995data,respectively.Other possible biases from the selection cuts on the measurement of the forward-backward asymmetry are negligible.This is verified by a Monte Carlo study which shows that events not selected for the asymmetry measurement,but inside thefiducial volume and withξ<15◦,do not have a different A FB value.The background from e+e−→τ+τ−(γ)events is found to have the same asymmetry as the signal and thus neither necessitates a correction nor causes a systematic uncertainty.The effect of the contribution from the two-photon process e+e−→e+e−µ+µ−,further reduced by the tighter acollinearity cut on the measured muon pair asymmetry,can be neglected.The forward-backward asymmetry of the cosmic ray muon background is measured to be−0.02±0.13using the events in the sideband of the distribution of closest approach to the interaction point. Weighted by the relative contribution to the data set this leads to corrections of−0.0007and +0.0003to the peak−2and peak+2asymmetries,respectively.On the peak this correction is negligible.The statistical uncertainty of the measurement of the cosmic ray asymmetry causes a systematic error of0.0001on the peak and between0.0003and0.0005for the peak−2and peak+2data sets.The systematic uncertainties on the measurement of the muon forward-backward asymmetry are summarised in Table9.In1993−94the total systematic error amounts to0.0008at the peak points and to0.0009at the off-peak points due to the larger contamination of cosmic ray muons.For the1995data the determination of systematic errors is limited by the number of events taken with the new detector configuration and the total error is estimated to be0.0015.In Figure15the differential cross sections dσ/dcosθmeasured from the1993−95data sets are shown for three different centre-of-mass energies.The data are corrected for detector acceptance and charge confusion.Data sets with a centre-of-mass energy close to m Z,as well as the data at peak−2and the data at peak+2,are combined.The data are compared to the differential cross section shape given in Equation3.The results of the total cross section and forward-backward asymmetry measurements in e+e−→µ+µ−(γ)are presented in Section10.8e+e−→τ+τ−(γ)Event SelectionThe selection of e+e−→τ+τ−(γ)events aims to select all hadronic and leptonic decay modes of the tau.Z decays into tau leptons are distinguished from other Z decays by the lower visible energy due to the presence of neutrinos and the lower particle multiplicity as compared to hadronic Z pared to our previous analysis[4]the selection of e+e−→τ+τ−(γ) events is extended to a larger polar angular range,|cosθt|≤0.92,whereθt is defined by the thrust axis of the event.Event candidates are required to have a jet,constructed from calorimetric energy de-posits[36]and muon tracks,with an energy of at least8GeV.Energy deposits in the hemisphere opposite to the direction of this most energetic jet are combined to form a second jet.The two jets must have an acollinearity angleξ<10◦.There is no energy requirement on the second jet.High multiplicity hadronic Z decays are rejected by allowing at most three tracks matched to any of the two jets.In each of the two event hemispheres there should be no track with an angle larger than18◦with respect to the jet axis.Resonant four-fermionfinal states with a high mass tau pair and a low mass fermion pair are mostly kept in the sample.The multiplicity cut affects only tau decays into three charged particles with the soft fermion close in space leading to corrections of less than1‰.If the energy in the electromagnetic calorimeter of thefirst jet exceeds85%,or the energy of the second jet exceeds80%,of the beam energy with a shape compatible with an electromagnetic shower the event is classified as e+e−→e+e−(γ)background and hence rejected. Background from e+e−→µ+µ−(γ)is removed by requiring that there be no isolated muon with a momentum larger than80%of the beam energy and that the sum of all muon momenta does not exceed1.5E b.Events are rejected if they are consistent with the signature of two MIPs.To suppress background from cosmic ray events the time of scintillation counter hits asso-ciated to muon candidates must be within±5ns of the beam crossing.In addition,the track in the muon chambers must be consistent with originating from the interaction point.In Figures16to19the energy in the most energetic jet,the number of tracks associated to both jets,the acollinearity between the two jets and the distribution of|cosθt|are shown for the1994data.Data and Monte Carlo expectations are compared after all cuts are applied, except the one under study.Good agreement between data and Monte Carlo is observed.Small discrepancies seen in Figure17are due to the imperfect description of the track reconstruction efficiency in the central chamber.Their impact on the total cross section measurement is small and is included in the systematic error given below.Tighter selection cuts must be applied in the region between barrel and end-cap part of the BGO calorimeter and in the end-cap itself,reducing the selection efficiency(see Figure19). This is due to the increasing background from Bhabha scattering.Most importantly the shower shape in the hadron calorimeter is also used to identify candidate electrons and the cuts on the energy of thefirst and second jet in the electromagnetic end-cap calorimeter are tightened to 75%of the beam energy.。
最终最大重投影误差 英文
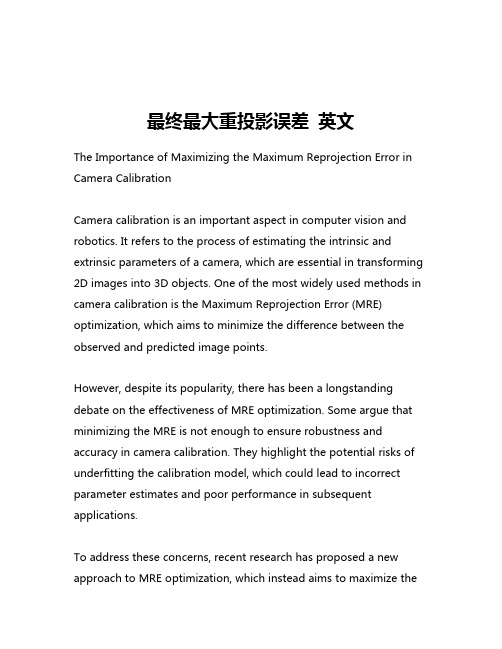
最终最大重投影误差英文The Importance of Maximizing the Maximum Reprojection Error in Camera CalibrationCamera calibration is an important aspect in computer vision and robotics. It refers to the process of estimating the intrinsic and extrinsic parameters of a camera, which are essential in transforming 2D images into 3D objects. One of the most widely used methods in camera calibration is the Maximum Reprojection Error (MRE) optimization, which aims to minimize the difference between the observed and predicted image points.However, despite its popularity, there has been a longstanding debate on the effectiveness of MRE optimization. Some argue that minimizing the MRE is not enough to ensure robustness and accuracy in camera calibration. They highlight the potential risks of underfitting the calibration model, which could lead to incorrect parameter estimates and poor performance in subsequent applications.To address these concerns, recent research has proposed a new approach to MRE optimization, which instead aims to maximize themaximum reprojection error. This involves finding the optimal parameter values that result in the largest possible MRE, subject to a certain level of constraint. The rationale behind this approach is that by emphasizing the effect of outliers, it can improve the robustness and accuracy of the calibration model, especially in cases where the data is noisy or corrupted.For instance, in a scenario where the camera captures images under varying lighting conditions, there may be significant variations in the observed image points, which could cause the MRE optimization to fail. However, by maximizing the maximum reprojection error, the calibration model can be more tolerant to nonlinearities and deviations, which can improve its overall performance.In conclusion, maximizing the maximum reprojection error is an important consideration in camera calibration. It offers a promising solution to the challenges associated with MRE optimization, by emphasizing the importance of outliers and nonlinearities in the data. As computer vision and robotics continue to evolve, it is likely that this approach will become increasingly relevant in ensuring robust and accurate camera calibration.。
2019年最新-Cosmic Strings and Superstrings宇宙字符串和超弦-精选文档
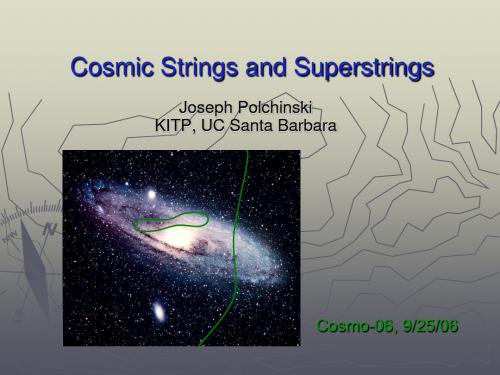
4-d picture: breakage of flux tube due to monopole-antimonopole pair production.
details of compactification.
• Strings with axion charge are confined.
• Strings with Aharonov-Bohm charges are absolutely stable.
Production of cosmic strings:
Example: gauge theory solitons. These solutions exist as topological defects in the Higgs field whenever a U(1) symmetry is broken:
our brane
Brane inflation:
An attractive model of inflation is that there were additional brane-antibrane pairs in the early universe. Their energy density induced inflation; subsequently they annihilated:
Instabilities of strings II
II. Some strings are ‘confined’ by a strong selfattraction:
cd模型的工作原理
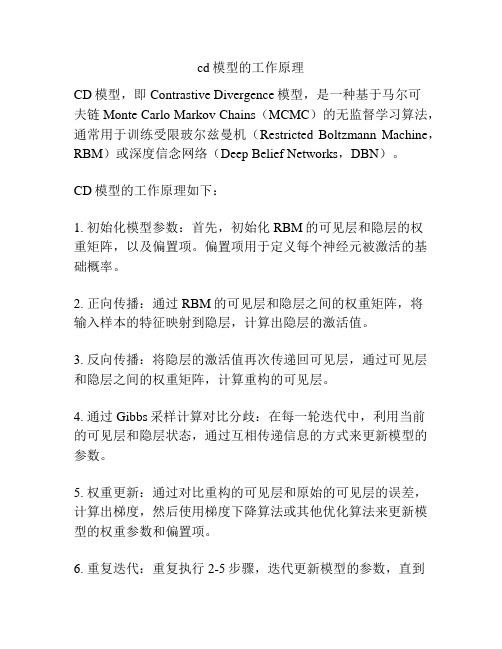
cd模型的工作原理
CD模型,即Contrastive Divergence模型,是一种基于马尔可
夫链Monte Carlo Markov Chains(MCMC)的无监督学习算法,通常用于训练受限玻尔兹曼机(Restricted Boltzmann Machine,RBM)或深度信念网络(Deep Belief Networks,DBN)。
CD模型的工作原理如下:
1. 初始化模型参数:首先,初始化RBM的可见层和隐层的权
重矩阵,以及偏置项。
偏置项用于定义每个神经元被激活的基础概率。
2. 正向传播:通过RBM的可见层和隐层之间的权重矩阵,将
输入样本的特征映射到隐层,计算出隐层的激活值。
3. 反向传播:将隐层的激活值再次传递回可见层,通过可见层和隐层之间的权重矩阵,计算重构的可见层。
4. 通过Gibbs采样计算对比分歧:在每一轮迭代中,利用当前
的可见层和隐层状态,通过互相传递信息的方式来更新模型的参数。
5. 权重更新:通过对比重构的可见层和原始的可见层的误差,计算出梯度,然后使用梯度下降算法或其他优化算法来更新模型的权重参数和偏置项。
6. 重复迭代:重复执行2-5步骤,迭代更新模型的参数,直到
达到预定的迭代次数或收敛条件。
通过这种方式,CD模型通过学习数据的分布特征,能够对输入数据进行无监督的特征学习和表示学习,进而可以用于生成样本和进行概率推断等任务。
- 1、下载文档前请自行甄别文档内容的完整性,平台不提供额外的编辑、内容补充、找答案等附加服务。
- 2、"仅部分预览"的文档,不可在线预览部分如存在完整性等问题,可反馈申请退款(可完整预览的文档不适用该条件!)。
- 3、如文档侵犯您的权益,请联系客服反馈,我们会尽快为您处理(人工客服工作时间:9:00-18:30)。
I.
INTRODUCTION
One of the standard methods of interpreting the growing body of evidence from supernovae [1] and other measurements that the expansion of the Universe is accelerating, is to assume the existence of a dark energy component and to model it using scalar fields (for a recent review see [2]). This links the expansion history of the Universe to theories of fundamental physics. For example, from this perspective the value of the potential at its minimum is the cosmological constant (CC). Since at this point there are many theoretical ideas about the form of the potential but none that particularly stands out, it would have been helpful if the data from cosmological measurements, such as supernovae Ia, CMB and various others, could provide hints about some generic features of the potential. Viable scalar field models of dark energy need to have potentials whose energy scale is about the critical density ∼ 10−12 eV4 , and typical scalar field masses about the Hubble mass m ∼ 10−33 eV. In such models typical scalar field variations are about Planck scale mp ∼ 1019 GeV, and typical time scales for such variations are about the Hubble time 1/H0 ∼ 1018 sec.
Distinguishing among Scalar Field Models of Dark Energy
Irit Maor, Ram Brustein
Department of Physics, Ben-Gurion University, Beer-Sheva 84105, Israel e-mail: irrit,ramyb@bgumail.bgu.ac.il
We show that various scalar field models of dark energy predict degenerate lu-
arXiv:hep-ph/0209203v1 18 Sep 2002
minosity distance history of the Universe and thus cannot be distinguished by supernovae measurements alone. In particular, models with a vanishing cosmological constant (the value of the potential at its minimum) are degenerate with models with a positive or negative cosmological constant whose magnitude can be as large as the critical density. Adding information from CMB anisotropy measurements does reduce the degeneracy somewhat but not significantly. Our results indicate that a theoretical prior on the preferred form of the potential and the field’s initial conditions may allow to quantitatively estimate model parameters from data. Without such a theoretical prior only limited qualitative information on the form and parameters of the potential can be extracted even from very accurate data.
2 Whether, and how well, it is possible to determine the parameters and form of potentials and the field’s dynamical history and future from data beyond such qualitative estimates has been addressed previously [3, 4, 5, 6, 7, 8, 9, 10, 11]. Weller and Albrecht [5] concluded that some potentials could be differentiated using SNAP-like data [12]. They approximated the equation of state (EOS) of each of the models, and showed by likelihood analysis that some of the models are distinguishable. Another approach is “reconstruction” [9, 10, 11]. Here one rewrites the potential as a function of the luminosity-distance (dL) and its derivatives, which are in turn functions of the redshift. The potential (and not the EOS) is approximated by a fitting function, and statistically tested against a set of accurate dL measurements. The efficiency of reconstruction depends on the accuracy of knowing the values of Ωm (this is needed also when one fits the EOS), and H0 (this is needed only for reconstruction). On general grounds we expect that scalar field potentials are less distinguishable than their corresponding EOS, because different potentials, with properly adjusted initial conditions for the field can produce very similar EOS. In previous papers [13, 14] we have found that supernovae (SN) measurements are limited as a probe of the dark energy EOS wQ , due to degeneracies. Specifically, it was shown that dL ’s corresponding to two different wQ ’s are degenerate if both EOS coincide at some point at a relatively low red shift, z ∗ (see also [15] and [16]). The purpose of the present analysis is to explore the implications of this degeneracy on the possibility to determine the scalar field potential. For a given functional form of potential, we would like to quantify the amount by which the parameters of the potential can be varied, and still be indistinguishable by accurate SN measurements. Our criteria for indistinguishability between two models is that their resulting dL ’s differ at most by 1% up to redshift z = 2, in accordance with the anticipated accuracy of future SN measurements. In addition, we would like to determine whether the functional form of the potential can be distinguished or constrained by data. We look for degeneracies among potentials using the following procedure: For a given class of potentials, we change the parameters as well as the initial values of the field, with the constraint that wQ at z ∗ remains unchanged. This results in models whose wQ cross at z ∗ . We know from our previous analysis that in this case the models tend to be degenerate. Then we evaluate numerically the differences in the dL’s of the models to verify this. There are additional sources of degeneracy that we do not consider here. In our procedure the value of the potential energy and the value of the kinetic energy remain unchanged.