20120426-Electrochimica Acta-Wang Huihui
高密度阳极铝电解槽电

第 54 卷第 2 期2023 年 2 月中南大学学报(自然科学版)Journal of Central South University (Science and Technology)V ol.54 No.2Feb. 2023高密度阳极铝电解槽电−热场耦合仿真研究魏兴国1,廖成志1,侯文渊1, 2,段鹏1,李贺松1(1. 中南大学 能源科学与工程学院,湖南 长沙,410083;2. 中北大学 能源与动力工程学院,山西 太原,030051)摘要:在铝电解槽中,阳极炭块内存在的气孔会降低炭块的导电和导热性能,并且增加炭渣,降低电流效率,导致炭耗和直流电耗升高。
通过浸渍工艺得到的高密度阳极可以有效地降低炭块的气孔率。
为了探究高密度阳极铝电解槽的电−热场变化和影响,基于ANSYS 软件建立高密度阳极铝电解槽的电−热场耦合计算模型。
研究结果表明:铝电解槽高密度阳极炭块的平均温度上升8.73 ℃,热应力增加,但形变量减小;侧部槽壳的平均温度下降28.59 ℃,热应力和形变量均降低,有利于保持槽膛内形稳定;热场变化主要与阳极炭块物性改变有关;槽电压降低49.16 mV ,主要与炭块物性改变和电解质电阻率降低有关;高密度阳极电流全导通时间缩短3.39 h ,可有效减弱换极产生的负面影响,阳极使用寿命可延长4 d ,炭耗降低10.3 kg/t ;铝电解槽反应能耗占比增加0.62%,电流效率提高1.69%,直流电耗降低270 kW·h/t 。
关键词:铝电解槽;高密度阳极;电−热场;耦合仿真中图分类号:TF821 文献标志码:A 文章编号:1672-7207(2023)02-0744-10Simulation study of electric-thermal field coupling in high-densityanode aluminum electrolyzerWEI Xingguo 1, LIAO Chengzhi 1, HOU Wenyuan 1, 2, DUAN Peng 1, LI Hesong 1(1. School of Energy Science and Engineering, Central South University, Changsha 410083, China;2. School of Energy and Power Engineering, North University of China, Taiyuan 030051, China)Abstract: In aluminum electrolytic cells, porosity in anode carbon blocks can reduce the electrical and thermal conductivity of the blocks and increase carbon slag, reduce current efficiency and lead to higher carbon consumption and DC power consumption. High-density anodes obtained by impregnation process can effectively reduce the porosity of carbon blocks. In order to investigate the electric-thermal field variation and the causes of influence in the high-density anode aluminum electrolyzer, a coupled electric-thermal field calculation model of收稿日期: 2022 −07 −11; 修回日期: 2022 −08 −20基金项目(Foundation item):国家高技术研究发展项目(2010AA065201);中南大学研究生自主探索创新项目(2021zzts0668)(Project(2010AA065201) supported by the National High-Tech Research and Development Program of China; Project (2021zzts0668) supported by the Independent Exploration and Innovation of Graduate Students in Central South University)通信作者:李贺松,博士,教授,博士生导师,从事铝电解研究;E-mail:****************.cnDOI: 10.11817/j.issn.1672-7207.2023.02.032引用格式: 魏兴国, 廖成志, 侯文渊, 等. 高密度阳极铝电解槽电−热场耦合仿真研究[J]. 中南大学学报(自然科学版), 2023, 54(2): 744−753.Citation: WEI Xingguo, LIAO Chengzhi, HOU Wenyuan, et al. Simulation study of electric-thermal field coupling in high-density anode aluminum electrolyzer[J]. Journal of Central South University(Science and Technology), 2023, 54(2): 744−753.第 2 期魏兴国,等:高密度阳极铝电解槽电−热场耦合仿真研究the high-density anode aluminum electrolyzer was established based on ANSYS software. The results show that the average temperature of the anode carbon block increases by 8.73 ℃ when the high-density anode is put on the tank, and the thermal stress increases but the deformation variable decreases. The average temperature of the side shell decreases by 28.59 ℃, and the thermal stress and deformation variable both decrease,which helps to protect the inner shape of the tank chamber stable. The change of the thermal field is mainly related to the change of the physical properties of the anode carbon block. The cell voltage decreases by 49.16 mV which is mainly related to the change of carbon block physical ploperties and the decrease of electrolyte resistivity, respectively. The reduction of 3.39 h in the full conduction time of high-density anode current can effectively reduce the negative effects of electrode change, and the anode service life can be extended by 4 d. The carbon consumption is reduced by 10.3 kg/t. The reaction energy consumption of aluminum electrolyzer is increased by 0.62%, the current efficiency is increased by 1.69%, and the DC power consumption is reduced by 270 kW·h/t.Key words: aluminum electrolyzer; high-density anode; electric-thermal field; coupling simulation作为铝电解槽的核心部件,阳极炭块在反应过程中被不断消耗,其品质直接影响着各项经济技术指标[1]。
Electrochimica Acta 132 (2014) 364–369
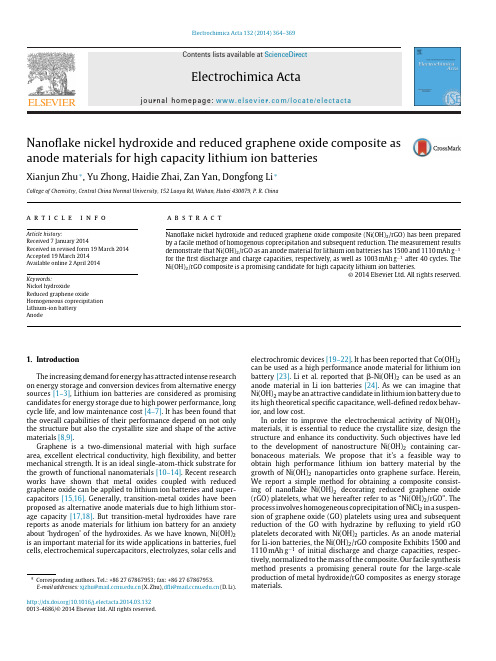
Electrochimica Acta 132(2014)364–369Contents lists available at ScienceDirectElectrochimicaActaj o u r n a l h o m e p a g e :w w w.e l s e v i e r.c o m /l o c a t e /e l e c t a c taNanoflake nickel hydroxide and reduced graphene oxide composite as anode materials for high capacity lithium ion batteriesXianjun Zhu ∗,Yu Zhong,Haidie Zhai,Zan Yan,Dongfong Li ∗College of Chemistry,Central China Normal University,152Luoyu Rd,Wuhan,Hubei 430079,P.R.Chinaa r t i c l ei n f oArticle history:Received 7January 2014Received in revised form 19March 2014Accepted 19March 2014Available online 2April 2014Keywords:Nickel hydroxideReduced graphene oxideHomogeneous coprecipitation Lithium-ion battery Anodea b s t r a c tNanoflake nickel hydroxide and reduced graphene oxide composite (Ni(OH)2/rGO)has been prepared by a facile method of homogenous coprecipitation and subsequent reduction.The measurement results demonstrate that Ni(OH)2/rGO as an anode material for lithium ion batteries has 1500and 1110mAh g −1for the first discharge and charge capacities,respectively,as well as 1003mAh g −1after 40cycles.The Ni(OH)2/rGO composite is a promising candidate for high capacity lithium ion batteries.©2014Elsevier Ltd.All rights reserved.1.IntroductionThe increasing demand for energy has attracted intense research on energy storage and conversion devices from alternative energy sources [1–3],Lithium ion batteries are considered as promising candidates for energy storage due to high power performance,long cycle life,and low maintenance cost [4–7].It has been found that the overall capabilities of their performance depend on not only the structure but also the crystallite size and shape of the active materials [8,9].Graphene is a two-dimensional material with high surface area,excellent electrical conductivity,high flexibility,and better mechanical strength.It is an ideal single-atom-thick substrate for the growth of functional nanomaterials [10–14].Recent research works have shown that metal oxides coupled with reduced graphene oxide can be applied to lithium ion batteries and super-capacitors [15,16].Generally,transition-metal oxides have been proposed as alternative anode materials due to high lithium stor-age capacity [17,18].But transition-metal hydroxides have rare reports as anode materials for lithium ion battery for an anxiety about ‘hydrogen’of the hydroxides.As we have known,Ni(OH)2is an important material for its wide applications in batteries,fuel cells,electrochemical supercapacitors,electrolyzes,solar cells and∗Corresponding authors.Tel.:+862767867953;fax:+862767867953.E-mail addresses:xjzhu@ (X.Zhu),dfli@ (D.Li).electrochromic devices [19–22].It has been reported that Co(OH)2can be used as a high performance anode material for lithium ion battery [23].Li et al.reported that -Ni(OH)2can be used as an anode material in Li ion batteries [24].As we can imagine that Ni(OH)2may be an attractive candidate in lithium ion battery due to its high theoretical specific capacitance,well-defined redox behav-ior,and low cost.In order to improve the electrochemical activity of Ni(OH)2materials,it is essential to reduce the crystallite size,design the structure and enhance its conductivity.Such objectives have led to the development of nanostructure Ni(OH)2containing car-bonaceous materials.We propose that it’s a feasible way to obtain high performance lithium ion battery material by the growth of Ni(OH)2nanoparticles onto graphene surface.Herein,We report a simple method for obtaining a composite consist-ing of nanoflake Ni(OH)2decorating reduced graphene oxide (rGO)platelets,what we hereafter refer to as “Ni(OH)2/rGO”.The process involves homogeneous coprecipitation of NiCl 2in a suspen-sion of graphene oxide (GO)platelets using urea and subsequent reduction of the GO with hydrazine by refluxing to yield rGO platelets decorated with Ni(OH)2particles.As an anode material for Li-ion batteries,the Ni(OH)2/rGO composite Exhibits 1500and 1110mAh g −1of initial discharge and charge capacities,respec-tively,normalized to the mass of the composite.Our facile synthesis method presents a promising general route for the large-scale production of metal hydroxide/rGO composites as energy storage materials./10.1016/j.electacta.2014.03.1320013-4686/©2014Elsevier Ltd.All rights reserved.X.Zhu et al./Electrochimica Acta132(2014)364–3693652.Experimental2.1.Synthesis of graphite oxideGraphite oxide was synthesized from natural graphite by a mod-ified Hummers method.Briefly,graphite powders(2g;500mesh, Sinopharm Chemical Reagent Co.,Ltd)were mixed,then put into concentrated H2SO4(96ml;98%)in an ice bath.Under vigorous stirring,KMnO4(6g;99.5%)was gradually added and the temper-ature of the mixture was kept below20◦C.After removing the ice bath,the mixture was stirred at35◦C in a water bath for18h.As the reaction progressed,the mixture became pasty with a brown-ish color.150ml H2O was then slowly added to the pasty mixture. Addition of water into the concentrated H2SO4medium generates large amounts of heat;therefore water should be added slowly and while keeping the mixture in an ice bath to maintain the tempera-ture below50◦C.After dilution with240ml H2O,5ml of30%H2O2 (Sinopharm Chemical Reagent Co.,Ltd)was added to the mixture, and the color of this diluted solution became a brilliant yellow. After continuously stirring for2h,the mixture wasfiltered and washed with10%HCl(aq)(250ml),then DI water and then anhy-drous ethanol to remove other ions.Finally,the resulting solid was dried under vacuum.2.2.Preparation of Ni(OH)2/rGO compositeThe nanoflake Ni(OH)2/rGO composite was prepared by homo-geneous precipitation and then subsequent reduction with hydrazine by refluxing.In a typical experiment,5mmol NiCl2 (0.65g;>98%)was dissolved in50ml water,150mmol urea(9.0g; 98%)was separately dissolved in50ml water,then urea and NiCl2 solutions were slowly and sequentially added to50ml of2mg ml−1 graphite oxide suspension under stirring.After exposure to ultra-sound from an ultrasonic bath for30min,the mixture was heated at90◦C for1.5h.When cooled to room temperature,0.5ml N2H4 (85%,Sinopharm Chemical Reagent Co.,Ltd)was added to the mix-ture while it was stirred.Then the mixture was refluxed at100◦C for 24h in an oil bath,in which the mixture color changed from black-brown to black.Then,the black mixture was collected byfiltration. After washing with DI water in an attempt to remove any excess hydrazine as well as other ions,the as-prepared product was dried at80◦C for8h under vacuum in order to obtain a Ni(OH)2/rGO com-posite.For comparison,Ni(OH)2without rGO was also synthesized using the same procedure.2.3.CharacterizationsThe structure of the as-prepared Ni(OH)2/rGO composite was characterized by X-ray diffraction(XRD,Cu K␣radiation;=0.15414nm)at the scan rate of2◦min−1in the2range of5 and80◦.Scanning electron microscopy(SEM)was performed using JSM-6700F(field emission gun;specimen chamber pressure of about10−5Pa;accelerating voltage5kV;working distance8mm). Transmission electron microscopy(TEM,JEM-2010FEF;200keV) was used to study the morphology and microstructure of the com-posites.Raman spectrum measurements were carried out using INVIA(RENISHAW,England)system with a514.5nm wavelength incident laser light.Thermal gravimetric analysis(TGA)was mea-sured with a SDT600apparatus using a heating rate of5◦C min−1 under20ml min−1offlow air from25to850◦C.2.4.Electrochemical characterizationsElectrochemical experiments were performed using2032coin-type cells.The working electrode consisted of95wt%as-prepared active material and5wt%polytetrafluoroethylene binder.ThemassFig.1.Scheme for making Ni(OH)2/rGO composite.of active materials in the electrode is about5mg,and the area of tested electrode is about2cm2( 16mm).The electrolyte was a solution of1M LiPF6in EC/DEC(1:1by volume)(purchased from Zhangjiagang Guotai-Huarong New Chemical materials Co.Ltd). Pure Li foil(Aldrich)was used as the counter electrode and the sep-arator was Celgard2300.The cells were discharged and charged galvanostatically in a potential window of0.005∼3.0V using a Land battery tester(China)at room temperature.3.Results and discussionAs shown in Fig.1,graphite oxide prepared by a modified Hum-mers method[25,26],was sonicated in water to form a suspension of GO platelets.For the synthesis of the Ni(OH)2/rGO composite, NiCl2was hydrolyzed in the GO suspension in the presence of urea at90◦C for1.5h in an oil bath.The molar ratio of NiCl2to urea was1:30.This step yielded a uniform Ni(OH)2particles coating on the surface of the GO platelets.During hydrolysis,urea releases hydroxyl ions slowly and uniformly in the suspension,resulting in the formation of Ni(OH)2as suggested by the following reactions: CO(NH2)2+3H2O→2NH4++CO2+2OH−(1)Ni2++2OH−→Ni(OH)2(2)Some of the Ni(OH)2particles connect together to form nanoflakes and likely anchor onto the surface of the GO platelets through oxygen-containing functional groups,such as hydroxyl, epoxyl,and carboxyl,but further work is indicated to elucidate the detailed chemical bonding,if any,at the surface.After the suspension was cooled to room temperature,a trace of hydrazine was added to the suspension under continuous stir-ring and the suspension is refluxed at100◦C for24h in an oil bath, which converts GO to rGO.As the as-obtained sample was charac-terized by XRD(Fig.2a),the main characteristic peaks of Ni(OH)2 appears,which agrees with JCPDS00-022-0444.For Raman spec-tra measurements(Fig.2b),the Raman spectrum at low incident laser energy shows the D and G characteristic peaks of rGO,while increasing the incident laser energy,the Raman spectrum exhibits only the characteristic peaks of NiO.It can be explained that rGO is burnt out and Ni(OH)2decomposes to NiO as Ni(OH)2→NiO+H2O at high incident laser energy in the air while rGO is protected by the covering of Ni(OH)2particles on the surface at low incident laser energy.Based on the results of XRD and Raman spectra,the as-prepared composite is composed of Ni(OH)2and rGO.By cal-culation from TGA result of the composite,the content of rGO is 23%.The morphology of the Ni(OH)2/rGO composite was observed by scanning electron microscopy as shown in Fig.3.Fig.3a and b show that Ni(OH)2/rGO composite consists of thin,crumpled366X.Zhu et al./Electrochimica Acta 132(2014)364–369Fig.2.(a)XRD patterns of (i)Ni(OH)2/rGO,(ii)Ni(OH)2,and (iii)JCPDS 22-0444.(b)Raman spectra of (i)Ni(OH)2/rGO at low incident laser energy,(ii)Ni(OH)2/rGO at high incident laser energy,and (iii)free Ni(OH)2.rGO platelets closely connected with each other to form a 3D network structure,and Ni(OH)2particles are distributed on the curved rGO platelets.The morphology of Ni(OH)2particles can be seen from TEM images shown in Fig.3c,which shows nanoflake structure of Ni(OH)2.From the HRTEM image of Fig.3d,a single nanoflake Ni(OH)2is composed of ∼5nm Ni(OH)2nanoparticles,which dispays a single-crystalline structure from the inlet image of Fig.3d.The lattice spacing of 0.74nm corresponds to the d spacing between adjacent (100)crystallographic planes of Ni(OH)2crystal [27].To measure the performance of the Ni(OH)2/rGO composite as an anode for Li ion batteries,the composite was mixed with poly-tetrafluoroethylene (PTFE)in a weight ratio of 95:5for preparing a working electrode,which is equivalent to Ni(OH)2:rGO:PTFE =73:22:5,and is practical for commercial battery anodes.Carbon black (CB)or other carbonaceous materials can increase the conductivity of the electrode when preparing the electrode for electrochemical measurement.However,they can also lower the weight specific capacity of the electrode.In our experiment,carbon black was not added to the electrode,in contrast to other studies [28–30].Fig.3.SEM images (a,b)and TEM images (c,d)of Ni(OH)2/rGO.X.Zhu et al./Electrochimica Acta 132(2014)364–369367Fig.4a shows the 1st ,2nd ,3rd ,10th and 40th discharge and charge curves of the Ni(OH)2/rGO composite at a current density of 100mA g −1between 0.005and 3.0V vs.Li/Li +.The Ni(OH)2/rGO composite delivers 1500and 1110mAh g −1specific capacity for the first discharge and charge,respectively,based on the total mass of the Ni(OH)2/rGO composite (the values are 1948and 1442mAh g −1,respectively,based on the mass of Ni(OH)2in the composite).During the first discharge,the potential decreases steeply from open circuit potential (3.07V)to 1.25V,and then a plateau region appears at 1.18V up to a capacity of 508mAh g −1,which is equivalent to a binding of 1.76Li per Ni(OH)2.Another slope is observed below 0.82V,yielding a total first discharge capacity of 1500mAh g −1,corresponding to a total binding of 5.19Li per Ni(OH)2.Correspondingly,the first charge capacity of 1110mAh g −1is equivalent to 3.84Li per Ni(OH)2.The sec-ond discharge and charge capacities are 1109.6and 1044mAh g −1,respectively.From the second cycle on,the discharge and charge curves are similar.Based on the result of discharge and charge for Ni(OH)2/rGO composite,it is suggested that the electrochemical reaction mech-anism of lithium with Ni(OH)2be as follows [23]:Ni (OH )2+2Lilithium insertionlithium extractionNi +2LiOH(3)To exactly confirm the mechanism,in situ XRD and 7LiNMR measurements of the relevant active phases in the electrochemical cycling process will be adopted in the further research.For the complete reduction of 2Li +Ni(OH)2 2LiOH +Ni,one would expect a maximum uptake of 2Li/Ni(OH)2(578mAh g −1).However,the value of the first discharge is 5.19Li,and that of the first charge 3.84Li for the Ni(OH)2/rGO composite,which is more than the theoretical value of 2Li per Ni(OH)2.What gives rise to this ‘excess capacity’?The excess capacity appears to orig-inate from electrolyte decomposition in the low-potential region,and thus perhaps the subsequent formation of an organic layer on the surface of the particles [31,32],as well as possible Li inser-tion/extraction (or simple decoration on open surfaces)of the rGO platelets.In the present study,it is suggested that Li inser-tion/extraction/decoration in rGO may play a major role in the overall electrochemical process and could be the primary reason for the excess capacity of the Ni(OH)2/rGO composite electrode.By comparison of the first cycles among Ni(OH)2/rGO,Ni(OH)2and rGO,the first charge capacity of 1110mAh g −1for Ni(OH)2/rGO is higher than that of 1088mAh g −1for free Ni(OH)2mixed phys-ically with carbon black,and that of 503mAh g −1for rGO itself,respectively.The results indicate that the Ni(OH)2/rGO composite has more lithium insertion/extraction/decoration sites than free Ni(OH)2or rGO.This is perhaps because the Ni(OH)2nanoflakes are anchored on the surface of the rGO platelets can act as spacers between the rGO platelets during discharging and charging,leading rGO in the composite to have a large surface area for storing Li,and meanwhile making the composite electrode to have a good conduc-tivity,which is favor to enhancing the electrochemical performance of the Ni(OH)2/rGO composite.Fig.4b shows the cycle performance of the Ni(OH)2/rGO com-posite at 100mA g −1in the potential range of 0.005and 3.0V.The discharge capacity of Ni(OH)2/rGO composite drops from 1500mAh g −1to 1109.6mAh g −1after the first cycle.However,the discharge capacity is gradually restored after 10cycles.The dis-charge capacity in the first,2nd ,10th ,20th ,30th and 40th cycles are 1500,1109.6,985.6,986.4,993.3,and 1003mAh g −1,respectively.A comparison indicates that the reversible capacity (∼1003mAh g −1after 40cycles at 100mA g −1)is higher than that of -Ni(OH)2@rGO previously reported (507mAh g −1after 30cycles at 200mA g −1)[24].The discharge capacity of Ni(OH)2/nickel foam fabricated by Ni et al.(∼0.59mAh cm −2at 100mA g −1)[33],and that of Ni(OH)2/Niby Tian et al.(∼0.82mAh cm −2at 50mA g −1)[34],are much smaller than the discharge capacity value of 2.51mAh cm −2at 100mA g −1after 40cycles obtained in our study.The phenomenon of an initial drop in capacity following by a gradual increase is well documented in the literature [35–37],which is attributed to some irreversible Li 2O generated in the dis-charge step.Nevertheless,in the present study,Li 2O comes not only from LiOH as 2LiOH Li 2O +H 2O and electrolyte degradation,but also partly from rGO due to the electrochemical reduction of oxygen-containing functional groups on the surface of rGO during the discharge step.We suggest that the electro-reduction reactions could be as follows:C COLi +CCLi 2O +(4)C HO Li +Li 2O+C +H 2(5)CLi 2O+CLi+HOOC+H 2+CO 2(6)On the other hand,due to the large surface area of the nanoflake Ni(OH)2/rGO composite,Li 2O is likely to result in the reversible formation and decomposition of a polymeric gel-like film on the large surface of the active nanoparticles as well as on the large surface of rGO platelets.Furthermore,this film can also prohibit the growth of active particles,leading to gradually increasing dis-charge and charge capacities based on the good conductivity of rGO platelets.The coulombic efficiency of the first cycle is 74%.After 4cycles,the columbic efficiency is more than 97%,indicating that the composite also has good capacity retention.The Ni(OH)2/rGO com-posite showed good rate performance as well (Fig.4c).The specific capacity was as high as 779mAh g −1even at a current density of 1600mA g −1.The high capacity and good cycling stability of the Ni(OH)2/rGO as an anode material is attributed to the intimate contact between the nanoflake Ni(OH)2and the rGO platelets.The uniform mixture and interaction between the nanoflake Ni(OH)2and rGO platelets can evidently accommodate the volume change of active particles during discharging and charging,and can prevent the aggregation of active particles and the restacking of rGO sheets,which likely also enhances cycle stability.In a control experiment,we synthesized free Ni(OH)2by the same method without any rGO.Although the free Ni(OH)2parti-cles has sphere-like morphology,which is different from that of nanoflake Ni(OH)2,its crystallinity is similar to that of the nanoflake Ni(OH)2.The result indicates that rGO can act as a template in the formation of nanoflake Ni(OH)2by homogeneous coprecipitation.The electrochemical performance of free Ni(OH)2mixed physically with carbon black was much worse than that of Ni(OH)2/rGO.At a current density of 100mA g −1,free Ni(OH)2had 1697mAh g −1and 1088mAh g −1for the first discharge and charge capacities,respec-tively,but after 40cycles,they decreased rapidly to 166mAh g −1and 161mAh g −1,respectively (Fig.4d).As comparison,pure rGO had 1590and 503mAh g −1for the first discharge and charge capac-ities,and further decreased to 240mAh g −1and 234mAh g −1after 40cycles,respectively.The comparison of the cycling performance among rGO,free Ni(OH)2and Ni(OH)2/rGO shows that the total specific capacity of Ni(OH)2/rGO is higher than the sum of free Ni(OH)2and rGO in the composite.This indicates a positive syner-gistic effect of nanoflake Ni(OH)2and rGO platelets in the composite for improving electrochemical performance.The cyclic voltammetric curves of the Ni(OH)2/rGO composite between 0.005and 3.0V (vs Li/Li +)are shown in Fig.4e.In the first cycle,a broaden reduction peak at ∼0.5V and a pronounced peak at 0.92V can be attributed to the formation of a solid electrolyte inter-face (SEI)on the electroactive materials,the reduction of Ni(OH)2368X.Zhu et al./Electrochimica Acta 132(2014)364–369Fig.4.Electrochemical performance of the Ni(OH)2/rGO composite.The specific capacities are based on the mass of the composite.(a)Discharge/charge profiles of Ni(OH)2/rGO at the current density of 100mA g −1.(b)Cycling performance of Ni(OH)2/rGO composite at the current density of 100mA g −1.(c)Rate capacity of Ni(OH)2/rGO composite between 0.005and 3.0V at different current densities.(d)Comparison of cycling performance among Ni(OH)2/rGO,pure Ni(OH)2and rGO in the potential range of 0.005∼3.0V at the current density of 100mA g −1.(e)Cyclic voltammograms of the Ni(OH)2/rGO composite at a scan rate of 0.1mV/s in the voltage range of 0.005∼3.0V.into Ni and LiOH,respectively.The CV curves of the 2nd and 3rd cycle are similar,whereas they are different from that of the first cycle.In the second cycle,the reduction peaks shift positively to 0.72and 1.69V,respectively,indicating the activation of the elec-trode.In the anodic scan,three oxidation peaks at about 1.12,1.40and 2.37V could be assigned to the decomposition of SEI and LiOH as well as the oxidation of Ni into Ni(OH)2.The peaks in the cyclic voltammetric curves are consistent with the plateaus or sloping potential ranges of charging and discharging profiles.The CV curves show good reproducibility,suggesting a high degree of reversibility for the electrochemical reactions.4.ConclusionsIn summary,a simple approach is developed to fabricate the composite composed of rGO platelets decorated with nanoflake Ni(OH)2.The Ni(OH)2/rGO has 1500and 1110mAh g −1for the first discharge and charge capacities,respectively.After 40cycles,it still has 1003mAh g −1capacity,which is much more than the theoretical capacity 372mAh g −1of graphite.It exhibits enhanced electrode performance in lithium ion battery.Our simple synthe-sis method can be readily adapted to prepare other composites in which rGO not only acts as a good conducting additive to supportX.Zhu et al./Electrochimica Acta132(2014)364–369369metal hydroxide nanoparticles,but also provides additional elec-trochemical active sites to further enhance their energy storage performance.AcknowledgementsWe are grateful for thefinancial support from the Natural Sci-ence Foundation of Hubei Province(No.2011CDB161)and the Scientific Research Foundation for the Returned Overseas Chinese Scholars,State Education Ministry(SRF for ROCS,SEM).Appendix A.Supplementary dataSupplementary data associated with this article can be found,in the online version,at /10.1016/ j.electacta.2014.03.132.References[1]A.S.Arico,P.Bruce,B.Scrosati,J.-M.Tarascon,W.van Schalkwijk,Nat.Mater.4(2005)366.[2]Y.-G.Guo,J.-S.Hu,L.-J.Wan,Adv.Mater.20(2008)2878.[3]M.Winter,R.J.Brodd,Chem.Rev.104(2004)4245.[4]H.S.Zhou,D.L.Li,M.Hibino,I.Honma,Angew.Chem.Int.Edit.44(2005)797.[5]D.W.Liu,B.B.Garcia,Q.F.Zhang,Q.Guo,Y.H.Zhang,S.Sepehri,G.Z.Cao,Adv.Funct.Mater.19(2009)1015.[6]A.Burke,J.Power Sources91(2000)37.[7]P.Simon,Y.Gogotsi,Nat.Mater.7(2008)845.[8]J.M.Ma,X.C.Duan,J.B.Lian,T.Kim,P.Peng,X.D.Liu,Z.F.Liu,H.B.Li,W.J.Zheng,Chem.Eur.J16(2010)13210.[9]J.Chen,D.H.Bradhurst,S.X.Dou,H.K.Liu,J.Electrochem.Soc.146(1999)3606.[10]S.Garaj,W.Hubbard,A.Reina,J.Kong,D.Branton,J.A.Golovchenko,Nature467(2010)190.[11]S.Stankovich,D.A.Dikin,G.H.B.Dommett,K.M.Kohlhaas,E.J.Zimney,E.A.Stach,R.D.Piner,S.T.Nguyen,R.S.Ruoff,Nature442(2006)282.[12]A.K.Geim,Science324(2009)1530.[13]X.S.Li,W.W.Cai,J.H.An,S.Kim,J.Nah,D.X.Yang,R.Piner,A.Velamakanni,I.Jung,E.Tutuc,S.K.Banerjee,L.Colombo,R.S.Ruoff,Science324(2009)1312.[14]ler,R.A.Outlaw,B.C.Holloway,Science329(2010)1637.[15]S.-M.Paek,E.Yoo,I.Honma,Nano Lett.9(2008)72.[16]D.Wang,D.Choi,J.Li,Z.Yang,Z.Nie,R.Kou,D.Hu,C.Wang,L.V.Saraf,J.Zhang,I.A.Aksay,J.Liu,ACS Nano3(2009)907.[17]P.Poizot,ruelle,S.Grugeon,L.Dupont,J.M.Tarascon,Nature407(2000)496.[18]W.Yao,J.Yang,J.Wang,L.Tao,Electrochim.Acta53(2008)7326.[19]P.Jeevanandam,Y.Koltypin,A.Gedanken,Nano Lett.1(2001)263.[20]V.Srinivasan,J.W.Weidner,J.Electrochem.Soc.147(2000)880.[21]C.Natarajan,H.Matsumoto,G.Nogami,J.Electrochem.Soc.144(1997)121.[22]J.He,H.Lindstrom,A.Hagfeldt,S.-E.Lindquist,J.Phys.Chem.B103(1999)8940.[23]Y.-S.He,D.-W.Bai,X.Yang,J.Chen,X.-Z.Liao,Z.-F.Ma,mun.12(2010)570.[24]B.Li,H.Cao,J.Shao,H.Zheng,Y.Lu,J.Yin,M.Qu,mun.47(2011)3159.[25]S.Stankovich,R.D.Piner,S.T.Nguyen,R.S.Ruoff,Carbon44(2006)3342.[26]W.S.Hummers,R.E.Offeman,J.Am.Chem.Soc.80(1958)1339.[27]L.Liu,Y.Li,S.M.Yuan,M.Ge,M.M.Ren,C.S.Sun,Z.Zhou,J.Phys.Chem.C114(2010)251.[28]H.Wang,L.-F.Cui,Y.Yang,H.Sanchez Casalongue,J.T.Robinson,Y.Liang,Y.Cui,H.Dai,J.Am.Chem.Soc.132(2010)13978.[29]S.M.Paek,E.Yoo,I.Honma,Nano Lett.9(2009)72.[30]G.M.Zhou,D.W.Wang,F.Li,L.L.Zhang,N.Li,Z.S.Wu,L.Wen,G.Q.Lu,H.M.Cheng,Chem.Mater.22(2010)5306.[31]S.R.Mukai,T.Hasegawa,M.Takagi,H.Tamon,Carbon42(2004)837.[32]W.B.Xing,J.R.Dahn,J.Electrochem.Soc.144(1997)1195.[33]S.Ni,X.Lv,T.Li,X.Yang,L.Zhang,Journal of Materials Chemistry A1(2013)1544.[34]J.Tian,Z.Xing,Q.Chu,Q.Liu,A.M.Asiri,A.H.Qusti,A.O.Al-Youbi,X.Sun,CrystEngComm15(2013)8300.[35]R.Dedryvere,ruelle,S.Grugeon,P.Poizot,D.Gonbeau,J.M.Tarascon,Chem.Mater.16(2004)1056.[36]S.Grugeon,ruelle,L.Dupont,J.M.Tarascon,Solid State Sci.5(2003)895.[37]Y.Yu,C.H.Chen,J.L.Shui,S.Xie,Angew.Chem.Int.Edit.44(2005)7085.。
ElectroActa-2010-LiMei
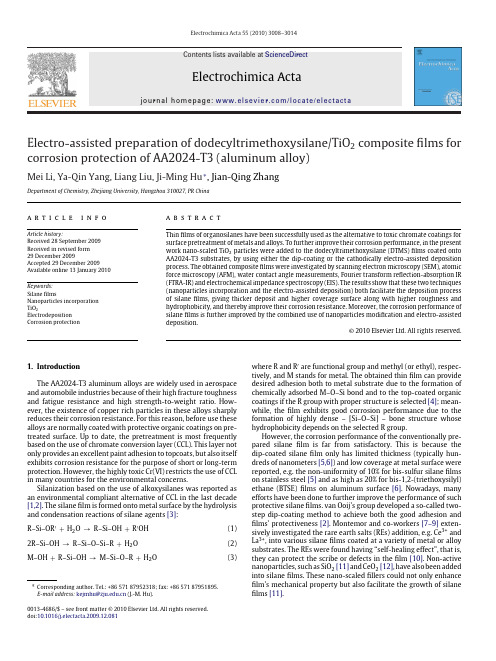
Electrochimica Acta 55 (2010) 3008–3014Contents lists available at ScienceDirectElectrochimicaActaj o u r n a l h o m e p a g e :w w w.e l s e v i e r.c o m /l o c a t e /e l e c t a c taElectro-assisted preparation of dodecyltrimethoxysilane/TiO 2composite films for corrosion protection of AA2024-T3(aluminum alloy)Mei Li,Ya-Qin Yang,Liang Liu,Ji-Ming Hu ∗,Jian-Qing ZhangDepartment of Chemistry,Zhejiang University,Hangzhou 310027,PR Chinaa r t i c l e i n f o Article history:Received 28September 2009Received in revised form 29December 2009Accepted 29December 2009Available online 13 January 2010Keywords:Silane filmsNanoparticles incorporation TiO 2Electrodeposition Corrosion protectiona b s t r a c tThin films of organosilanes have been successfully used as the alternative to toxic chromate coatings for surface pretreatment of metals and alloys.To further improve their corrosion performance,in the present work nano-scaled TiO 2particles were added to the dodecyltrimethoxysilane (DTMS)films coated onto AA2024-T3substrates,by using either the dip-coating or the cathodically electro-assisted deposition process.The obtained composite films were investigated by scanning electron microscopy (SEM),atomic force microscopy (AFM),water contact angle measurements,Fourier transform reflection-absorption IR (FTRA-IR)and electrochemical impedance spectroscopy (EIS).The results show that these two techniques (nanoparticles incorporation and the electro-assisted deposition)both facilitate the deposition process of silane films,giving thicker deposit and higher coverage surface along with higher roughness and hydrophobicity,and thereby improve their corrosion resistance.Moreover,the corrosion performance of silane films is further improved by the combined use of nanoparticles modification and electro-assisted deposition.© 2010 Elsevier Ltd. All rights reserved.1.IntroductionThe AA2024-T3aluminum alloys are widely used in aerospace and automobile industries because of their high fracture toughness and fatigue resistance and high strength-to-weight ratio.How-ever,the existence of copper rich particles in these alloys sharply reduces their corrosion resistance.For this reason,before use these alloys are normally coated with protective organic coatings on pre-treated surface.Up to date,the pretreatment is most frequently based on the use of chromate conversion layer (CCL).This layer not only provides an excellent paint adhesion to topcoats,but also itself exhibits corrosion resistance for the purpose of short or long-term protection.However,the highly toxic Cr(VI)restricts the use of CCL in many countries for the environmental concerns.Silanization based on the use of alkoxysilanes was reported as an environmental compliant alternative of CCL in the last decade [1,2].The silane film is formed onto metal surface by the hydrolysis and condensation reactions of silane agents [3]:R–Si–OR +H 2O →R–Si–OH +R OH (1)2R–Si–OH →R–Si–O–Si–R +H 2O (2)M–OH +R–Si–OH →M–Si–O–R +H 2O(3)∗Corresponding author.Tel.:+8657187952318;fax:+8657187951895.E-mail address:kejmhu@ (J.-M.Hu).where R and R are functional group and methyl (or ethyl),respec-tively,and M stands for metal.The obtained thin film can provide desired adhesion both to metal substrate due to the formation of chemically adsorbed M–O–Si bond and to the top-coated organic coatings if the R group with proper structure is selected [4];mean-while,the film exhibits good corrosion performance due to the formation of highly dense –[Si–O–Si]–bone structure whose hydrophobicity depends on the selected R group.However,the corrosion performance of the conventionally pre-pared silane film is far from satisfactory.This is because the dip-coated silane film only has limited thickness (typically hun-dreds of nanometers [5,6])and low coverage at metal surface were reported,e.g.the non-uniformity of 10%for bis-sulfur silane films on stainless steel [5]and as high as 20%for bis-1,2-(triethoxysilyl)ethane (BTSE)films on aluminum surface [6].Nowadays,many efforts have been done to further improve the performance of such protective silane films.van Ooij’s group developed a so-called two-step dip-coating method to achieve both the good adhesion and films’protectiveness [2].Montemor and co-workers [7–9]exten-sively investigated the rare earth salts (REs)addition,e.g.Ce 3+and La 3+,into various silane films coated at a variety of metal or alloy substrates.The REs were found having “self-healing effect”,that is,they can protect the scribe or defects in the film [10].Non-active nanoparticles,such as SiO 2[11]and CeO 2[12],have also been added into silane films.These nano-scaled fillers could not only enhance film’s mechanical property but also facilitate the growth of silane films [11].0013-4686/$–see front matter © 2010 Elsevier Ltd. All rights reserved.doi:10.1016/j.electacta.2009.12.081M.Li et al./Electrochimica Acta55 (2010) 3008–30143009A bigger breakthrough in silanefilm’s preparation process occurred after Mandler’s group proposed an electrochemically assisted technique(EAT)[13].The cathodically generated OH−ions catalyze the condensation reactions among silanols themselves (reaction(2))and between silanols and metal substrate(reaction (3)),only in the small electrolyte volume near the electrode sur-face.The localized alkalization may overcome the shortcoming of a high pH bulk silane solution,where the hydrolysis rate is less and the condensation rate is high,leading to the insufficient generation of silanols and dramatic loss in solution stability.It was reported that[14,15]the silanefilms prepared by the EAT are thicker,less porous,better organized and more uniform,if compared to the dip-coatedfilms.As a result,thesefilms present better corrosion performance.Nevertheless,there are only few works[14–20]using EAT to prepare silanefilms for the purpose of corrosion protection.Our previous publication[21]might be thefirst work report-ing the combination of EAT and nanoparticles addition to prepare highly corrosion-resistive silanefilms.The results showed that both the nano-scaled silica incorporation and EAT can improve the formation of dodecyltrimethoxysilane(DTMS)films and their protectiveness.More importantly,the combined techniques can further improve the above-mentioned credits.In the present paper, we have prepared TiO2nanoparticles-incorporated DTMSfilms by the EAT,to further confirm the combined effect of these two tech-niques on the formation and protective properties of silanefilms. The work is focused on,apart from the corrosion performance,the physico-chemical properties evaluation of the incorporatedfilms, such as surface morphology,film thickness and the hydrophobicity.2.ExperimentalThe AA2024-T3aluminum alloy substrate(Southwest Alu-minum,Chongqing,China)was cut to coupons(3cm×6cm)and then mechanically polished with emery paper(600grit)prior tofilm deposition.After polishing,the samples were thoroughly rinsed with home-made surfactant-based low alkaline cleaner,and finally washed with deionized(DI)water and then blow-dried with warm air.All samples were kept in a desiccator for at least24h before use.The titanium dioxide nanoparticles(20±5nm,as mea-sured by the producer),with a purity≥99.9%,were purchased from Chemat Chemical(Xia’men,China).Silane agent(DTMS: CH3(CH2)11Si(OCH3)3,95%)was purchased from Zhejiang Chemi-cal Industry Research Institute(Hangzhou,China).The blank silane solution contains5vol.%silane agent dissolved in75/25(v/v) ethanol/water mixed solvent(pH4.5adjusted by acetic acid).Then, the obtained solution was pre-hydrolyzed at35◦C for48h form-ing a sol–gel precursor.The TiO2-containing silane solution was obtained by adding appropriate amount of nanoparticles(0,20,40, 70,100,150,200mg/L,respectively)into the blank solution,and the mixed solutions were further stirred for1h to make sure the nanoparticles dispersed well.The electrodeposition was performed by using three-electrode cell,with the saturated calomel electrode (SCE)as the reference and a platinum plate(2.0cm×2.0cm)as the counter.The silanefilms were deposited at the open-circuit potential(OCP,approximately−0.3V/SCE),corresponding to the dip-coating process,and at various cathodic potentials(i.e.−0.6,−0.8,−1.0,−1.2and−1.4V/SCE,respectively).The deposition was conducted for200s,after which samples were taken out and blow-dried with nitrogen to remove any excess liquid,finally cured at 100◦C for1h at air in an oven.The surface morphology of silanefilms wasfirst examined on a SIRIONfield emission scanning electron microscopy(SEM)pro-duced by FEI Co.Ltd.(USA),and then the3D images were obtained by an atomic force microscopy(AFM,SPI3800N,Seiko Instruments Inc.,Chiba,Japan).The thickness of the silanefilm was measured on a variable-angle spectroscopic ellipsometer(model VASE;J.A. Woollam Inc.,Lincoln,NE,USA)at incident angles of65◦,70◦and 75◦within a wavelength range of800–1100nm.To minimize the measuring error originated from the incompletely smooth sur-face of aluminum alloys,thefilms used for thickness measurement were deposited onto a commercial conductive mirror-like smooth silicon substrate(P-doped,(111)-oriented,8–12 cm resistivity, 0.525mm thickness)purchased from Ningbo QL Electronics Co., Ltd.(China).Detailed processes about the substrate pretreatment can refer to the reference[22].The thickness of the silanefilms was measured at three different areas,and was calculated from the ellipsometric parameters,,by which thefilm thickness and refractive index were automaticallyfitted using a Cauchy model. Thefilms’static water contact angles were measured in the air by JC2000X A static dropping contact angle measuring instrument (Shanghai,China)with high speed CCD camera for imagecapture.Fig.1.AFM scan(100m×100m)of un-modified(a and b)and TiO2nanoparti-cles(40mg/L)-modified(c and d)DTMSfilms.(a and c)Dip-coatedfilms;(b and d)films deposited at−0.8V.Left column:2D topgraph;right column:3D scans.3010M.Li et al./Electrochimica Acta55 (2010) 3008–3014DI water drops with the volume of∼15L were used.Contact angle was calculated by the average of measured angles at10randomly selected spots.Fourier transform reflection-absorption IR(FTRA-IR) was measured at silanefilms coated on AA2024-T3substrates.The measurement was carried out on a Nicolet470spectrophotometer (Thermo Nicolet,USA)with incidence angle of80◦normal to the surfaces of the specimens,spectral resolution of4cm−1,number of scans of32and un-treated bare aluminum alloy as the background.The corrosion performance of silanefilm-coated aluminum alloy electrodes was evaluated by electrochemical impedance spec-troscopy(EIS).The measurement was carried out at30◦C after the immersion of working electrode(∼3.0cm2of exposed area)into the corrosive solution for30min on a M273model potentiostat (Princeton Applied Research,USA)combined with a M5210model lock-in amplifier(Princeton Applied Research,USA).The measured frequency was selected from120kHz to0.01Hz with an ac exci-tation amplitude of10mV at open-circuit potential.The testing electrolyte was a3.5wt.%NaCl aqueous solution prepared with DI water.The same three-electrode cell was used as described above.3.Results and discussion3.1.Physico-chemical characterization offilmsThe AFM images clearly show the differences in morphol-ogy for various groups offilm.The dip-coated DTMS-onlyfilm cannot effectively cover the whole substrate area,which is evi-denced from the visible polishing scratches on the underlying aluminum alloy substrate(Fig.1a).After applying a cathodic poten-tial of−0.8V(SCE),these scratches are almost entirelyfilled by the electrodeposited material(Fig.1b),indicating the existence of electro-assisted base-catalyzed gelation.Modifying with a cer-tain amount of TiO2nanoparticles gives a nano-structured surface silanefilm(Fig.1c).This columnar-like structure becomes clearer when the compositefilm is obtained by cathodic electrodeposi-tion(Fig.1d).A similar columnar-like nano-structure has been reported in silica nanoparticles-doped Ce-silanefilms[23].The 3D views(right column of thefigure)provide further informa-tion of the surface roughness of the deposits.A homogenous and compact deposit is obtained for pure DTMSfilm prepared by elec-trodeposition(Fig.1b).These merits(high homogeneity and high compactness)of electrodeposited silanefilms have already been described in van Ooij and co-workers’[15]and our previous works [16,17].The nano-structured micro morphology is much more clearly seen from the3D views of nanoparticles-modifiedfilms (Fig.1c and d).Moreover,the better arranged nano-structure is obtained for electrodeposited compositefilms(Fig.1d).The root-mean-squared roughness(RMS)of the dip-coated pure DTMSfilm(RMS=65.5nm,Fig.1a)is slightly increased when applying a cathodic potential of−0.8V(RMS=89.7nm,Fig.1b), whereas the TiO2-modified deposits are significantly higher and continuously increases with the enhanced nanoparticles content therein(shown in Fig.2,RMS=131.3,154.0and313.6for dip-coated compositefilms obtained from precursors containing40, 100and150mg/L TiO2nanoparticles,respectively).Furthermore, the RMS of the compositefilms is in larger extent enhanced by the cathodic electrodeposition process(inset in Fig.2),as compared to un-modified deposits.The increased roughness of thefilms indicates the facilita-tion infilms growth.The TiO2nanoparticles seem to act as the nuclei for further growing of thefilm,resulting in significant increase in thefilm roughness.Shown in Fig.3are the mea-sured andfitted spectroscopic ellipsometry«spectra at some typical silanefilms.It is shown that thefilm thickness increases from427.3±16.8nm for the dip-coated pure DTMS deposit to 552.5±11.4nm when thefilm is deposited under a negative potential of−0.8V(vs.SCE),suggesting theelectro-generatedFig.2.Roughness recorded by wet mode AFM of thefilm as a function of TiO2content in precursors(dip-coating method)and of deposition potential(inserted plot,for compositefilms).M.Li et al./Electrochimica Acta 55 (2010) 3008–30143011Fig.3.Spectroscopic ellipsometry «spectra taken at 65◦(1),70◦(2)and 75◦(3)for DTMS film loaded with 100mg/L TiO 2.Dashed green lines:experimental data;solid red lines:fitted results.(For interpretation of the references to color in this figure legend,the reader is referred to the web version of the article.)base-catalyzed gelation for the electrodeposited films [14].The thickness of the films can also be increased by adding proper amount of TiO 2nanoparticles (e.g.538.9±18.7nm for DTMS/TiO 2(100mg/L)),and further be significantly improved by cathodic elec-trodeposition (e.g.707.9±20.2nm for DTMS/TiO 2(100mg/L)film deposited at −0.8V).The improved growth of silane films by incor-porating proper amount of nanoparticles,e.g.silica,has already been reported in the previous reports [11,21].The improved effect on the gelation of silane films,by either the electrodeposition technique or the nanoparticles incorporation,is also evidenced by the Fourier transform reflection-absorption IR (FTRA-IR)measurements.Fig.4presents the FTRA-IR spectra of four different typical deposits on aluminum alloy substrates.The peaks at 2338and 2359cm −1are originated from O C O asymmetric stretching vibration,a result of the contamination of the atmo-sphere.The figure shows that the characteristic absorption of all the silane films under the investigation appears at ∼1145cm −1,which is attributed to the –Si–O asymmetric stretching in –Si–O–Si–[24],as a result of the condensation reactions (Eq.(2))among silanols.This absorption peak is found to obviously increase either after the cathodic deposition (if compare curve 2with 1for the pure DTMS films,or curve 4with 3for DTMS/TiO 2composite films)or after the nanoparticles modification (if compare curve 3withFig.4.FTRA-IR spectra of dip-coated (1and 3)and electrodeposited (2and 4)DTMS-only (1and 2)and DTMS/TiO 2composite (3and 4)films.Electrodeposition potential:−0.8V;TiO 2content in the precursor:100mg/L.1for dip-coated films,or curve 4with 2for electrodeposited films).This phenomenon clearly suggests the improved growth of silane film by both the electrodeposition and the nanoparticles incorpo-ration.It is also interesting to observe that an additional absorption peak at ∼1070cm −1is appeared at the electrodeposited pure DTMS film (curve 2)or TiO 2-doped composite films (curves 3and 4).This broaden peak was considered as the formation of metal–O–Si bonds in the metal/silane film interfacial layer [25],further indicating the positive effect of these two techniques on the formation of more protective silane films.The ease of organosilane films formation under electrodeposition and (or)nanoparticles incorporation may also be evidenced from the different peak absorption intensities at 1460cm −1,which is assigned to the ␦(CH 3)/␦(CH 2)vibrations [26]originated from the dodecyl group in DTMS molecule.The intensity of this absorption peak is significantly increased by either apply-ing cathodic potential (see curves 2and 4with respect to curves 1and 3,respectively)or doping the nanoparticles (see curves 3and 4with respect to curves 1and 2,respectively).Nevertheless,loading an excessive amount of nanoparticles gives a heterogeneous film surface (see Fig.5b for dip-coated DTMS/150mg/L TiO 2film).A large number of white agglomerates with their sizes ranged from 300to 600nm,which are identified by EDS as Ti-and oxygen-rich,are appeared on the top of film.These agglomerates cannot be entirely covered by silane deposits (Fig.5b),but the smaller agglomerates in the composite deposits with lower content of TiO 2nanoparticles are completely involved into the silane films (Fig.5a for dip-coated DTMS/100mg/L TiO 2film).On the other hand,as already reported in our previous pub-lications [16,17],the excessive negative potentials may lead to the intensive generation of hydrogen bubbles at electrode surface via water decomposition,and thereby leave a large number of pores and cracks in the films (see Fig.5c for DTMS/100mg/L TiO 2film deposited at −1.2V).The hydrophobic nature of the silane films is characterized by the contact angle measurements.The contact angle does not change much for the various films under investigation,because its value is mainly determined by the chemistry of the functional group in the silane films (dodecyl group in the present work).Nevertheless,the small but noticeable differences can still be found among these var-ious films.Fig.6shows that the hydrophobicity of DTMS films can be improved by loading a proper amount of TiO 2nanoparticles,in spite of the hydrophilic property of the nanoparticles them-selves.As the TiO 2content increases,the contact angle of the films increases firstly and then decreases.The improved hydrophobic-ity after doping a proper amount of nanoparticles agrees well with the characteristic morphologies,as well as the testing results of ellipsometry and IR of the composite deposits as mentioned above,that is they are more uniform,thicker,and in larger coverage.In addition,the nano-structured surface (see Fig.1c and d)of the nanoparticles-modified DTMS films may be more hydrophobic.The reduced hydrophobicity of heavily TiO 2-loaded composite films (e.g.150mg/L TiO 2)can be attributed to the exposed hydrophilic TiO 2agglomerates on the film surface due to the serious aggre-gation of nanoparticles (see Fig.5b).Inset in the figure indicates that the films hydrophobicity can be improved via electrodeposi-tion process at the proper cathodic potentials,which could also be explained by the higher uniformity,larger thickness and cov-erage of the electrodeposited films.The defects,such as pores and cracks,in the electrodeposited films obtained at excessively nega-tive potentials provide channels for water absorption,and thereby decrease the films hydrophobicity.3.2.Protective performance of silane filmsElectrochemical impedance spectroscopy (EIS)has been com-monly used to evaluate the corrosion performance of protective3012M.Li et al./Electrochimica Acta55 (2010) 3008–3014Fig.5.SEM images of dip-coated DTMS/(100mg/L)TiO 2(a)and DTMS/(150mg/L)TiO 2(b)films and electrodeposited DTMS/(100mg/L)TiO 2film (c).Electrodeposition potential:−1.2V.On the right bottom:EDS analysis on the whole surface and white dot-only area of image b,respectively.silane films on cold rolled steel [27,28],galvanized steel [28,29]and aluminum alloy [28,30]substrates.Fig.7shows the Bode plots of dip-coated DTMS/TiO 2composite films-treated 2024-T3panels in NaCl solution.The curves of bare 2024-T3are also presented in the figure as the reference.Only one broad time constant,which is a result of the over-lapping of two electrochemical processes occurred in the inner and outer oxide layers [31],is observed at the bare alloy (Fig.7b).According to Lee and Pyun [32,33],who have investigated the corrosion behaviors of pure aluminum in Cl −ion-contained aqueous solution,the high-frequency relaxation cor-responds to the Cl −ion-incorporated oxide layer which is formed during the induction time for pit initiation;while the intermediate-frequency relaxation is a result of inner oxide layer.After treated with silane films,significantly higher phase angles are exhibited in the high-frequency range (Fig.7b),due to the presence of protec-tive thin films.Moreover,the high-frequency phase angles increase when the films are modified with TiO 2nanoparticles (Fig.7b).The impedance modulus plots also show that comparing to that of the un-treated bare alloy the impedance of silane-coated samples is significantly increased (Fig.7a),suggesting that the silanefilmsFig.6.Contact angle of DTMS/TiO 2composite films.(a)Influence of TiO 2nanoparticles concentration in the sol solution on the dip-coated films;(b)influence of deposition potential on electrodeposited films.M.Li et al./Electrochimica Acta55 (2010) 3008–30143013Fig.7.Bode plots in3.5wt.%NaCl solution of bare2024-T3(square)and the alloy electrodes dip-coated with DTMSfilms loaded with0mg/L(circle),40mg/L(left-triangle),100mg/L(up-triangle),150mg/L(down-triangle)and200mg/L(diamond) TiO2nanoparticles.play an important role for corrosion protection of aluminum alloys. Fig.7a also shows that modifying with various content of TiO2 nanoparticles basically improves the protectiveness of DTMSfilms, as indicated from the increased impedance values.The highest impedance values are found at100mg/L of nanoparticles incor-poration.Detailed discussion on impedance data analysis on the basis of equivalent electric circuit(ECC)is beyond the range of the present work.One canfind intensive information in numeric datafitting of silanefilm-coated systems by selecting proper ECCs in our[18] and other group’s[4,25,34,35]previous publications.For the pur-pose of simplicity,the low-frequency impedance module(|Z|lf) is used here to quantitatively evaluate the protectiveness of un-doped and nanoparticles-doped silanefilms.|Z|lf reflects the sum of resistance of the resistive elements in the electric circuit if the frequency is selected as small as possible(theoretical value is0Hz). For silanefilm-treated metal or alloy system,|Z|lf commonly com-prises the solution resistance(R s),film resistance(R f)and charge transfer resistance(R ct)occurring at the substrate/electrolyte inter-face.Fig.8a clearly shows that the impedance value increases and then decreases when the content of doped nanoparticles increases. |Z|lf reaches the highest value at100mg/L TiO2concentration,sug-gesting the highest corrosion resistance of DTMS/TiO2composite film with this specific content.This is in good agreement with the result of contact angle measurement(Fig.6),and also is con-sistent with the morphology and thickness characterizations.As mentioned above,the nanoparticles incorporation results in an improved growth of silanefilms(Figs.1and2,and thickness data by elliposometry).But the excessive amount of TiO2doping gives seri-ous aggregation among nanoparticles(Fig.5b).These hydrophilic clusters cannot be entirely covered by DTMS deposits,and thereby exposes to thefilm surface.In this sense,the corrosion perfor-mance of DTMSfilms cannot be further enhanced by excessively incorporating nanoparticles.After applying cathodic deposition potentials onto TiO2-modified DTMSfilms,the corrosion resistance is further improved. Fig.8b shows the deposition potential-dependent|Z|lf of DTMS/TiO2(100mg/L)compositefilms as measured in NaCl solu-tion.The impedance values of electrodeposited pure DTMSfilms are also provided in thefigure as a reference.The corrosion perfor-mance of DTMSfilms shows a bell shape as a function of deposition potential,reaching the maximum at the deposition potential of −0.8V.Similar result has already been reported and discussed in our previous works[16,17],where some other silanefilms,e.g.bis-1,2-[triethoxysilyl]ethane(BTSE),vinyltrimethoxysilane(VTMS) and methyltrimethoxysilane(MTMS)films,all perform the high-est corrosion resistance when electrodeposited at this“critical potential”(−0.8V).The enhanced performance below the critical potential was believed as a result of base-catalyzed gelation due to the alkalization of electrolyte near the electrode surface under the cathodic polarization,whereas,the decreased corrosion resis-tance up the critical potential can be explained by the decreased compactness of obtainedfilms(e.g.pores and cracks)probably due to the attacking of formedfilms by tiny hydrogen bubbles at excessively negative potentials.Although the hydrogenbubblingFig.8.Low-frequency impedance modules(|Z|lf)of silanefilm deposited aluminum alloys in3.5%NaCl solution.(a)Dip-coated compositefilms as a function of TiO2 nanoparticles concentration in precursors.(b)Electrodeposited pure DTMSfilms and100mg/L TiO2-doped DTMSfilms as a function of deposition potential.|Z|lf is read at 0.01Hz.3014M.Li et al./Electrochimica Acta55 (2010) 3008–3014is hard to be visible due to very low current densities during the deposition(typically in the order of magnitude of micro-amperes), the cathodic voltammetric curves did suggest the occurrence of hydrogen evolving when the deposition potential is negative than −1.06V/SCE[16,17].Fig.8b clearly shows that the protective prop-erties of the electrodeposited compositefilms are obviously better than those of electrodeposited pure DTMSfilms,although their change trends with the variation of deposition potential are similar. The merit of the combined use of nanoparticles incorporation and cathodic electrodeposition in silanefilm’s preparation mainly lies on the facilitated effect onfilms growth by the individual factor as presented from the morphology,thickness and chemical character-ization.We have reported the similar results on electrodeposited silica-doped DTMSfilms[21].But up to date,it is still unclear why the nanoparticles incorporation can benefit the growth of silane films.Further work needs to be considered to understand better the above-mentioned issue.4.ConclusionsWe have prepared composite DTMS/TiO2films by the electro-chemically assisted technique(EAT)for corrosion protection of AA2024-T3alloys.Results show that incorporating the nano-scaled particles and applying the EAT both can obtain silane deposits with higher thickness and higher coverage and roughness surface.The obtainedfilms present higher hydrophobicity and better perfor-mance for corrosion protection.The above-mentioned credits can be further improved by the combined use of these two techniques (nanoparticles modification and electro-assisted deposition).AcknowledgementsThis work was supported by the National Natural Science Foundation of China(No.50871101)and the Chinese Universi-ties Scientific Fund.The authors also gratefully acknowledge the financial support from the State Key Laboratory for Corrosion and Protection.References[1]W.J.van Ooij,T.Child,Chemtech28(1998)26.[2]V.Subramanian,W.J.van Ooij,Corros54(1998)204.[3]G.Tesoro,Y.Wu,in:K.L.Mittal(Ed.),Silanes and Other Coupling Agents,VSP,Utrecht,NL,1992,p.215.[4]D.Zhu,W.J.van Ooij,Corros.Sci.45(2003)2177.[5]D.Zhu,W.J.van Ooij,Electrochim.Acta49(2004)1113.[6]A.Franquet,H.Terryn,J.Vereecken,Surf.Interface Anal.36(2004)681.[7]M.E.Montemor,M.G.S.Ferreira,Electrochim.Acta52(2007)6976.[8]M.G.S.Ferreira,R.G.Duarte,M.F.Montemor,A.M.P.Simoes,Electrochim.Acta49(2004)2927.[9]M.G.S.Ferreira,A.M.Cabral,W.Trabelsi,R.Serra,M.F.Montemor,M.L.Zhelud-kevich,Corros.Sci.48(2006)3740.[10]M.L.Zheludkevich,R.Serra,M.F.Montemor,M.G.S.Ferreira,-mun.7(2005)836.[11]Vignesh Palanivel,Danqing Zhu,W.J.van Ooij,.Coat.47(2003)384.[12]M.F.Montemor,R.Pinto,M.G.S.Ferreira,Electrochim.Acta54(2009)5179.[13]R.Shacham,D.Avnir,D.Mandler,Adv.Mater.11(1999)384.[14]M.Sheffer,A.Groysman,D.Mandler,Corros.Sci.45(2003)2893.[15]J.S.Gandhi,W.J.van Ooij,J.Mater.Eng.Perform.13(2004)475.[16]J.M.Hu,L.Liu,J.Q.Zhang,C.N.Cao,Electrochim.Acta51(2006)3944.[17]J.M.Hu,L.Liu,J.Q.Zhang,C.N.Cao,.Coat.58(2007)265.[18]J.M.Hu,L.Liu,J.Q.Zhang,C.N.Cao,Chem.J.Chin.Univ.(Chin.Ed.)27(2006)1121.[19]W.M.Zhang,J.M.Hu,Acta Metall.Sin.(Chin.Ed.)42(2006)295.[20]S.Z.Ding,L.Liu,J.M.Hu,J.Q.Zhang,C.N.Cao,Scripta Mater.59(2008)297.[21]L.Liu,J.M.Hu,J.Q.Zhang,C.N.Cao,Electrochim.Acta52(2006)538.[22]X.Gong,H.Yang,L.L.Han,C.Y.Gao,Langmuir24(2008)13925.[23]L.M.Palomino,P.H.Suegama,I.V.Aoki,M.F.Montemor,H.G.De Melo,Corros.Sci.51(2009)1238.[24]D.Q.Zhu,W.J.van Ooij,.Coat.49(2004)42.[25]W.J.van Ooij,D.Zhu,Corrosion57(2001)413.[26]Y.S.Li,Y.Wang,T.Tran,A.Perkins,Spectrochim.Acta Part A61(2005)3032.[27]L.Fedrizzi,F.J.Rodriguez,S.Rossi,F.Deflorian,R.D.Magi,Electrochim.Acta46(2001)3715.[28]G.P.Sundararajan,W.J.van Ooij,Surf.Eng.16(2000)315.[29]W.Trabelsi,L.Dhouibi,E.Triki,M.G.S.Ferreira,M.F.Montemor,Surf.Coat.Technol.192(2005)284.[30]M.Khobaib,L.B.Reynolds,M.S.Donley,Surf.Coat.Technol.140(2001)16.[31]I.V.Aoki,M.C.Bernard,S.I.C.d.Torresi,C.Deslouis,H.G.d.Melo,S.Joiret,B.Tribollet,Electrochim.Acta46(2001)1871.[32]W.J.Lee,S.I.Pyun,Electrochim.Acta45(2000)1901.[33]W.J.Lee,S.I.Pyun,Electrochim.Acta44(1999)4041.[34]A.Franquet,C.L.Pen,H.Terryn,J.Vereecken,Electrochim.Acta48(2003)1245.[35]A.Cabral,R.G.Duarte,M.F.Montemor,M.L.Zheludkevich,M.G.S.Ferreira,Cor-ros.Sci.47(2005)869.。
张紫薇李增增英语文献原版

Electrochimica Acta 108 (2013) 867–875Contents lists available at ScienceDirectElectrochimicaActaj o u r n a l h o m e p a g e :w w w.e l s e v i e r.c o m /l o c a t e /e l e c t a c taDiffusion mechanism of lithium ions in LiNi 0.5Mn 1.5O 4H.Seyyedhosseinzadeh,F.Mahboubi ∗,A.AzadmehrDepartment of Mining and Metallurgical Engineering,Amirkabir University of Technology,Hafez Avenue,P.O.Box 15875-4413,Tehran,Irana r t i c l ei n f oArticle history:Received 8March 2013Received in revised form 1July 2013Accepted 2July 2013Available online 16 July 2013Keywords:LiNi 0.5Mn 1.5O 4Diffusion mechanism Diffusion constant Activation energy Li-ion batterya b s t r a c tLiNi 0.5Mn 1.5O 4is suitable for electrochemical applications as an active material.This material has capa-bility for high rate application in Li-ion battery.In this research,the diffusion mechanism of Li ions in LiNi 0.5Mn 1.5O 4was studied by chronoamperometry technique and computer simulation (ab ini-tio and Fickian approach).According to the results,two bulk diffusion constants were calculated for LiNi 0.5Mn 1.5O 4during intercalation or deintercalation.At low concentration of Li ions in LiNi 0.5Mn 1.5O 4,the diffusion constant was about 10−9cm 2/s and for other Li ions concentration,it was about 10−11cm 2/s.In addition,the diffusion constant at the surface of LiNi 0.5Mn 1.5O 4was estimated 10−8cm 2/s by a semi empirical model.So,the diffusion constant of Li ions in LiNi 0.5Mn 1.5O 4has been estimated in the range of 10−8to 10−11cm 2/s.© 2013 Elsevier Ltd. All rights reserved.1.IntroductionThere are several crystal structures which can be used as active materials in Li-ion battery,yered,spinel and olivine like frame-works.The LiNi 0.5Mn 1.5O 4is spinel one and it is a suitable candidate for cathode of Li-ion battery.The LiNi 0.5Mn 1.5O 4spinel shows two different space groups (Fd 3m or P 4332)depending on Ni ordering in the lattice [1].It was confirmed by the X-ray diffraction (XRD)data that face-centered spinel (Fd 3m )transforms into primitive simple cubic (P 4332)structure by annealing process at 700◦C [2].The dif-ference of these two space groups is in distribution of Mn and Ni atoms in crystal structure of LiNi 0.5Mn 1.5O 4.The LiNi 0.5Mn 1.5O 4has a disordered distribution (Fd 3m ),where Li ions occupy the tetrahe-dral (8a)sites,Mn or Ni ions randomly occupy the octahedral (16d)sites and O ions are located at (32e)sites.In the ordered P 4332phase,Mn and Ni ions order on octahedral sites in a 3:1ratio (i.e.Ni atoms in 4b sites,Mn atoms in 12d sites,and O atoms in 8c and 24e sites according to the notations of Wyckoff position)[3–5].It was reported (by first principle calculation)that there is no intermediate phases between LiNi 0.5Mn 1.5O 4and Li 0Ni 0.5Mn 1.5O 4[6].It was also proved that LiMn 2O 4has better structural stability by Ni alloying due to the lower bond length of Mn O in the pres-ence of Ni.The Mn O and Mn(Ni)O bond length is 1.823and 1.800,respectively (by Raman scattering)[7].Rigid Spinel is better to pre-vent the insertion of the foreigner species instead of Li ions into it from electrolyte [8].In addition,it would be important to specify∗Corresponding author.Tel.:+982164542967;fax:+982166405846.E-mail address:Mahboubi@aut.ac.ir (F.Mahboubi).how the presence of Ni atoms would affect the overall movement of Li ions through the lattice of LiNi 0.5Mn 1.5O 4by affecting activation energies of diffusion.Quantum mechanics first principle calculation (ab initio)is a reliable computational method to calculate the activation energy for atomic diffusion.This computational method is successfully working for cutting edge technology/science [9],i.e.to design active materials for Li-ion batteries [10,11].In addition,other com-putational approaches (at mesoscale and marcoscale)are mostly relevant to assess how physical phenomena take place during de/intercalation of ions in Li-ions batteries.In general,the calcula-tion of flux (removal and insertion)of Li ions in active materials of Li-ion battery needs to consider different (by orders of magnitude)physical scales [12],e.g.more refine length/time scale at interface of active material and electrolyte in comparison to the bulk diffusion [13].It is not inequitable to claim that atomistic quantum based (ab initio)approach is more efficient to evaluate governing phys-ical mechanisms than the other computational methods at higher length scales.But atomistic calculations seem more difficult than other methods and they are also limited to small systems.Never-theless,performing simulation at any desired length scale would be helpful to predict and discover governing physical mechanisms.There are several coarse grain approaches based on the thermody-namic principles with attention to the de/intercalation phenomena [13–16].In addition,there are extensive ab initio quantum based models (atomistic approach)by considering a small part of Li-ion battery,i.e.active material or electrolyte [17–22].For LiNi 0.5Mn 1.5O 4,there are several published researches on total energy calculation,activation energy/barrier and diffusion coefficient of Li ions by computational and experimental methods0013-4686/$–see front matter © 2013 Elsevier Ltd. All rights reserved./10.1016/j.electacta.2013.07.034868H.Seyyedhosseinzadeh et al./Electrochimica Acta 108 (2013) 867–875Fig.1.The used algorithm of ab initio calculation for estimation of activation energy of Li ions for diffusion in the bulk of Li 8Ni 4Mn 12O 32.[6,23–26].Although there are some expressions on activation ener-gies of diffusion for accessible directions in LiNi 0.5Mn 1.5O 4,but it is still quite limited.In previous researches on activation energies of Li ions in LiNi 0.5Mn 1.5O 4,the diffusion direction with lowest acti-vation energy has been considered as the dominated one without considering the zigzag path of diffusion through octahedral and tetrahedral sites during diffusion.In this research,the diffusion mechanism of Li ions in LiNi 0.5Mn 1.5O 4was studied by chronoam-perometry and simulation (ab initio and Fickian approach)for more comprehensive description of diffusion of Li ions in LiNi 0.5Mn 1.5O 4.2.Experimental methods2.1.Fabrication of LiNi 0.5Mn 1.5O 4LiNi 0.5Mn 1.5O 4powders were synthesized via solid-state method.Appropriate amounts of MnO 2,Ni 2O 3and Li 2CO 3powders were mixed with ball milling for 10h.This planetary ball milling facility was used to activate powders.Subsequently,the mixedprecursor powders were calcinated in electric furnace at 800◦C for 5h and were cooled in air.2.2.Characterization of active materialX-ray diffraction (XRD)using CuK ␣radiation with wave length 0.154nm,Fourier Transform Infra-Red spectroscopy (FTIR)and scanning electron microscope (SEM)were used to characterize and assess the synthesized active material.Autolab PGSTAT30was used to measure the current versus time at constant voltage (chronoam-perometry)in active materials during deintercalation/charging (a two-electrode setup was used).The pure graphite was used as the reference electrode and cathode was connected to the working electrode.2.3.Assembly of batteryTo study the Li ions transportation in active material experi-mentally,0.06g of active material (LiNi 0.5Mn 1.5O 4)in powderformFig.2.Framework of multiscale model based on Fickian approach.H.Seyyedhosseinzadeh et al./Electrochimica Acta108 (2013) 867–875869Fig.3.The algorithm of multiscale model based on Fickian approach.without conductive material(i.e.carbon black)was poured in a small Al cap(current collector)as a cathode.And pure graphite was used as the anode.1M LiClO4in EC/DMC(1:1)was used as the electrolyte.The assembly of this cell was performed under Ar controlled atmosphere in a container.3.Frameworks of modelsIt was actually two separate models,first,ab initio model on cal-culating activation energy for Li ions during diffusion through bulk of LiNi0.5Mn1.5O4(and subsequent description of diffusion constant870H.Seyyedhosseinzadeh et al./Electrochimica Acta 108 (2013) 867–875Fig.4.An illustration of stochastic boundary condition at the interface of active material andelectrolyte.Fig.5.SEM images of LiNi 0.5Mn 1.5O 4.Fig.6.FTIR (a)and XRD (b)analysis of synthesized LiNi 0.5Mn 1.5O 4powders.by Arrhenius equation)and second,semi empirical model (multi-scale model:Fickian approach coupled with atomistic stochastic approach)for estimating the diffusion constant of Li ions at the surface of this activematerial.Fig.7.Hartree–Fock pseudopotential of atoms [29].3.1.Ab initio approachThis model was run in real physical space.Like any numerical methods,physical space must be discretized into some grids.The model used a cube with 0.81nm in each side as a domain of simula-tion.This domain was discretized by eight millions grids as meshes.The size of this cube is in accordance to the size of a supper cell of Li 8Ni 4Mn 12O 32.The total energy of an atomic system is composed of the kinetic energy of electrons,exchange–correlation energy (electrons quantum interaction),ion–ion interaction (Madelung energy),electron–electron coulomb interaction and pseudopoten-tial (valance electrons and core’s ions interaction plus the effect of inner electrons).Hartree–Fock pseudopotential [27]was used for capturing the effects of inner electrons and core’s ions poten-tial on valance electron cloud.In addition,local density approach (LDA)has been used to calculate the exchange–correlation energy.Despite the many successes of LDA theory,it is often claimed that this method is useless for strongly correlated materials (electron)[28].GGA +U method is proposed for strongly correlated materials [29,30].But the use of LDA or GGA has no effect on final results for calculating the activation energy [31].Finally,finite difference time domain was used to solve the Schrodinger equation [32].The overall strategy of present model has been shown in Fig.1.3.2.Fickian approachA single microscale particle of active material was considered in contact with electrolyte.The shape of the particle was estimatedH.Seyyedhosseinzadeh et al./Electrochimica Acta108 (2013) 867–875871Fig.8.Wave function for2s orbital. from SEM image of powders.To perform/run simulation,wholemodel must be discretized.The discreted model has106numbersof regular cubic meshes(with200nm length in side of each cubicmesh).Fig.2shows the framework of the model and its meshes.Thereby,there is the same mesh size for governing equations ofLi ionsflux both in electrolyte and active material;that is to say,there would be a difference between their time scales according tothe numerical stability condition.Instead of a proposed stochasticboundary condition,other used boundaries are common periodicboundary condition.The general algorithm of the model has beenshown in Fig.3.The proposed stochastic boundary condition workswith atoms,that is,this is an atomic approach.So,it has a new timescale different from the two time scales of atomic diffusion in activematerial and electrolyte.Tofind out an approximate time scale inused stochastic concept,following formula(Eq.(1))was used:D interface=1W ˇ2 →Stochastic time scale=ˇ2WD interface(1)Fig.9.Exchange–correlation energy for wave function in Fig.8.Fig.4shows an illustration of the used stochastic boundarycondition.ˇand W are related to the atomic configuration inLiNi0.5Mn1.5O4crystal.By having parameters in Eq.(1),D interface(the diffusion coefficient at the interface of active materialand Fig.10.Atomic distribution in Li7Ni4Mn16O32superlattice along with calculated pseudowave function in gray scale.The distance of the two adjacent slices is0.1nm.872H.Seyyedhosseinzadeh et al./Electrochimica Acta108 (2013) 867–875Fig.11.Spatial form of movement of lithium ion in three different paths. electrolyte)can be calculated.As the summation,the model pre-dicts the passing current by time evolution during charging.By regulating the calculated data by experimental results on charging current,stochastic time step can be determined and subsequently the introduced diffusion coefficient at the interface of active mate-rial and electrolyte would be specified.4.Results and discussionFig.5shows the SEM image of synthesized LiNi0.5Mn1.5O4parti-cles.The FTIR and XRD analysis of LiNi0.5Mn1.5O2have been shown in Fig.6.XRD and FTIR results confirmed the spinel LiNi0.5Mn1.5O4. Positions of Peaks in XRD and FTIR results are well adapted to the LiNi0.5Mn1.5O4spinel.The LiNi0.5Mn1.5O4spinel,depending on Niordering in the lattice,shows two different space groups(Fd3mFig.12.Calculated activation energies for three paths which have been shown in Fig.11.Fig.13.The chronoamperometry graph(current–time−0.5)of charging or during deintercalation of Li ions from LiNi0.5Mn1.5O4structure.or P4332).Although it is hard to detect these two space groups from each other by conventional XRD method,it was confirmed by the X-ray diffraction(XRD)data that face-centered spinel(Fd3m) transforms into primitive simple cubic(P4332)structure by anneal-ing process at700◦C[2].Hence,the simple cubic(P4332)structure was predicted for the space group of synthesized LiNi0.5Mn1.5O4 powders according to the used calcination temperature at800◦C in this research.In addition,by accepting the simple cubic(P4332) structure for synthesized LiNi0.5Mn1.5O4powders,some noisy like XRD patterns could be appointed as peaks such as(210),(211) and(220)peaks.Finally,this synthesized LiNi0.5Mn1.5O4powders were used for electrochemical tests as an active material in a cell. Along with electrochemical tests,two models were used forfinding out the diffusion mechanisms of Li ions in LiNi0.5Mn1.5O4.First model wasfirst principle calculation(ab initio model).This ab initio model uses a simple method to calculate the total energy of systems in real atomic space.For each atom(Li,O,Mn and Ni),a spherical symmetric pseudopotential was applied.Final potential is the summation of pseudopotential of all atoms in the model.Fig.7 shows the pseudopotential curve for atoms in Atomic Rydberg Unit (ARU/Ry).The same approach was used to define exchange–correlation energy.This term of energy is a function of local wave function, that is,this function is updated for every time step by new cal-culated wave function during simulation.Fig.8shows the wave function,which was defined on guesswork.This is a wave func-tion for2s orbital of Li atom.The wave function in Fig.8was used to draw the curve in Fig.9,which is shown the development of exchange–correlation potential in outward direction from the core of Liatom.Fig.14.Spiral diffusion path of Li ion though path3.H.Seyyedhosseinzadeh et al./Electrochimica Acta108 (2013) 867–875873In this model,the calculated wave function is pseudowave func-tion and it does not reflect the actual wave function of atoms.But the result is reliable on total energy.Fig.10shows the atomic arrangements in Li7Ni4Mn12O32superlattice with P4332crystal structure.According to the atomic arrangement in Li7Ni4Mn12O32 superlattice with P4332crystal structure,three directions or paths were checked for diffusing Li ions via them.These mentioned three paths have been spatially shown in Fig.11.Paths1and3have also shown in Fig.10by marking*and**.The difference between these two paths(1and3)is the connection of the nearest Ni ion to the Li ion when this Li ion is in the octahedral site during diffusion (Fig.11).In Fig.10,the movement of starred Li ion from slice6to the starred position in slice5and then to the starred vacancy posi-tion in slice4is related to the diffusion of Li ion along path1.And in thisfigure,the movement of double starred Li ion in slice2to the double starred position in slice3and then to the starred vacancy position in slice4is related to the diffusion of Li ion along path3.The activation energies have been calculated for these three mentioned paths by the proposed ab initio model.As it is obvi-ous,path2is not a proper candidate for diffusion of Li ion in Li7Mn16O32superlattice due to very high activation energy(Fig.12). Hence,the paths1and3are the most possible diffusion directions in Li7Ni4Mn12O32framework with24.18kJ/mol and39.14kJ/mol activation energies(Fig.12).So,the two possible passes for Li ion diffusion in LiNi0.5Mn1.5O4 have been confirmed by ab initio calculation.In ref.[24],it has been explained that10−9cm2/s diffusion constant would be achiev-able at low concentration of Li ions in Li X Ni0.5Mn1.5O4.It means that the diffusion mechanism of Li ions in LiNi0.5Mn1.5O4would Fig.15.Electrical potential around active material calculated from Poisson’s equa-tion.be changed during de/intercalation.This subject has been checked by chronoamperometry(Fig.13).As it was obvious,the graph (current–time−0.5;time is in second)of charging has different slope during deintercalation of Li ions from LiNi0.5Mn1.5O4structure.The overall diffusion mechanism of Li ion in this material would be described by considering the graph(Fig.13)in two separate regions. In region1,two mechanisms are controlling the produced current of the Li-ion cell(charges at the surface of active materials and dein-tercalation of Li ion by diffusion).As the curve in region2is more linear than region1,it might be true to consider the dominated overall mechanism is controlled by diffusion of Li ions for producing electrons in this region.In addition,it is obvious that thediffusion Fig.16.Concentration of Li ions in electrolyte after two seconds of charging at4.5V.874H.Seyyedhosseinzadeh et al./Electrochimica Acta 108 (2013) 867–875Fig.17.Estimated interfacial diffusion coefficient.coefficient in region 2is higher than in region 1as the slope of curve in region 2is higher than the slope of curve in region 1.To explain the diffusion mechanisms of Li ions in bulk of LiNi 0.5Mn 1.5O 4related to these two regions in Fig.13,two simple models have been considered.For region 1,the concentration of Li ions in active materials is not low,consequently all atomic posi-tions for diffusion of Li ions through path 1(the lowest activation energy of diffusion)are not always accessible.So,Li ions would dif-fuse through path 3(the path with higher activation energy than path 1)sometimes,that is,Li ions would diffuse through both paths (1and 3)according to their accessibility.For describing the over-all diffusion mechanism of Li ion in active materials in region 1,LiNi 0.5Mn 1.5O 4has been considered as a composite of these two passes.According to equation 2,the diffusion of Li ions can be esti-mated in the superlattice of Li 7Ni 4Mn 12O 32.By inserting 1012for Debye frequency (ϑ)and 0.28nm for length of Li ions hopping (a)and w =4(w is the number of octahedral sites around a Li ion in the tetrahedral site),the diffusion coefficient of paths 1and 3will be 2.89×10−9cm 2/s and 1.99×10−12cm 2/s.D =ϑa 2wexp−Q RT(2)Overall diffusion constant would be achieved by considering the Li 7Ni 4Mn 12O 32as a composite of the two paths (1and 3)for Li ions diffusion.Volume fraction of the two paths is the same (1:1).So,D composite =0.5D path 1+0.5D path 3It is good to notice that this calculated diffusion constant is related to the hopping of a Li ion from a tetrahedral site to the other nearest tetrahedral one via an octahedral site,that is,we cannot report these results as the overall diffusion coefficient of Li ions in Li 7Ni 4Mn 12O 32lattice because of neglecting the zigzag path of Li ions in Li 7Ni 4Mn 12O 32during diffusion in region 1.So,it still needs more correction for calculating the overall diffusion coefficient ofLi 7Ni 4Mn 12O 32.As the successful diffusion needs two jumps (First:from a tetrahedral site to octahedral one –Second:from an octahe-dral site to tetrahedral one),so the overall possible vacant positions for jumping are 8×4(4is the number of octahedral sites around a tetrahedral site and 8is the number of tetrahedral sites around an octahedral site).So,the geometrical probability of a successful jump of a Li ion from a tetrahedral to the nearest same one is 1/(8×4),which must be multiply to the D composite for calculating the overall diffusion of Li ion in Li 7Ni 4Mn 12O 32lattice.By this final modifica-tion,the zigzag movement during diffusion is applied to calculate the overall diffusion coefficient of Li 7Ni 4Mn 12O 32.According to mentioned subject the overall diffusion constant was calculated 4.51×10−11cm 2/s.This calculated result is consistent with pub-lished experimental results [6,26].This composite model was for region 1and the diffusion path of Li ion would be zigzag in LiNi 0.5Mn 1.5O 4.But in region 2(the low concentration of Li in LiNi 0.5Mn 1.5O 4),Li ions prefer to diffuse through lowest activation energy direction because of availability more vacant site for hoping a Li ion.Hence,Li ion diffuses through path 3and the overall diffusion coefficient in this region would be at the level of 10−9cm 2/s which was calculated 2.89×10−9cm 2/s.The diffusion path in this case would not be zigzag like region 1.In region 2,if Li ions prefer to diffuse through just path 3,the overall diffusion path would be spiral in one direction (Fig.14).So,Li ion moves forward in a direction with spiral movement.In addition,the diffusion constant at the surface of this active material was also estimated by a multiscale model for understand-ing the overall diffusion mechanism of Li ions in LiNi 0.5Mn 1.5O 4.A multi-scale model was developed to predict transportation of Li ions with main objective on interface of active materials and electrolyte.The calculated potential and Li ions distribution in elec-trolyte according to the framework in Fig.2have been shown in Figs.15and 16.These calculated results are measured after two seconds of charging.Finally,the model predicted 7.6×10−8cm 2/sH.Seyyedhosseinzadeh et al./Electrochimica Acta108 (2013) 867–875875as the diffusion constant at the surface of LiNi0.5Mn1.5O4 (Fig.17).5.ConclusionAn electrochemical test(chronoamperometry)and computer simulations(ab initio and Fickian approach)were used to estimate the overall diffusion mechanism of Li ion in LiNi0.5Mn1.5O4.The highlighted features of the present research are as follows:1.In LiNi0.5Mn1.5O4spinel with P4332crystal structure,it was con-firmed that just two directions are the possible corridors for Li ions to diffuse.The activation energies for these possible paths were calculated24.18and39.14kJ/mol.2.According to the calculated activation energies for diffusion ofLi ions in the spinel of Li7Ni4Mn12O32and by using Arrhenius form of diffusion equation,the calculated diffusion coeffi-cient for the two mentioned paths were2.89×10−9cm2/s and1.99×10−12cm2/s.3.By chronoamperometry test(at different concentration of Li ionin active material),two different mechanisms were explained for diffusion of Li ions in bulk of LiNi0.5Mn1.5O4.i.Low concentration of Li ions in LiNi0.5Mn1.5O4:Li ions preferto diffuse through lowest activation energy direction(with 10−9cm2/s diffusion constant).The diffusion path in this case would be spiral.ii.High and intermediate concentration of Li ions in LiNi0.5Mn1.5O4:By considering Li7Ni4Mn12O32as a composite of the two paths for diffusion of Li ions(with2.89×10−9cm2/s and1.99×10−12cm2/s diffusion constants),the overall dif-fusion constant was calculated4.51×10−11cm2/s,which hasa good consistency with experimental results.The path ofdiffusion would be zigzag.4.The diffusion coefficient of Li ions for jumping through theinterface of LiNi0.5Mn1.5O4and electrolyte was estimated by a multiscale model.The estimated value for this diffusion coeffi-cient was7.6×10−8cm2/s.References[1]C.M.Julien,F.Gendron,A.Amdouni,M.Massot,Lattice vibrations of materialsfor lithium rechargeable batteries.VI:ordered spinels,Materials Science and Engineering B130(2006)41.[2]S.H.Park,S.-W.Oh,S.H.Kang,I.Belharouak,K.Amine,Y.-K.Sun,Compara-tive study of different crystallographic structure of LiNi0.5Mn1.5O4−␦cathodes with wide operation voltage(2.0–5.0V),Electrochimica Acta52(2007) 7226.[3]T.-F.Yi,Y.Xie,M.-F.Ye,L.-J.Jiang,R.-S.Zhu,Y.-R.Zhu,Recent developmentsin the doping of LiNi0.5Mn1.5O4cathode material for5V lithium-ion batteries, Ionics17(2011)383.[4]G.Q.Liu,L.Wen,Y.M.Liu,Spinel,LiNi0.5Mn1.5O4and its derivatives as cath-odes for high-voltage Li-ion batteries,Journal of Solid State Electrochemistry 14(2010)2191.[5]N.Amdouni,K.Zaghib,F.Gendron,A.Mauger,C.M.Julien,Structure and inser-tion properties of disordered and ordered LiNi0.5Mn1.5O4spinels prepared by wet chemistry,Ionics12(2006)117.[6]H.Xi,Y.S.Meng,L.Lu,G.Ceder,Electrochemical properties of nonstoichiomet-ric LiNi0.5Mn1.5O4−␦thin-film electrodes prepared by pulsed laser deposition, Journal of the Electrochemical Society154(2007)A737.[7]Y.Wei,K.-B.Kim,G.Chen,Evolution of the local structure and electrochemicalproperties of spinel LiNi X Mn2−X O4(0≤X≤0.5),Electrochimica Acta51(2006) 3365.[8]J.B.Goodenough,A.Manthiram,B.Wnetrzewski,Electrodes for lithium batter-ies,Journal of Power Sources4344(1993)269.[9]A.Jain,G.Hautier,C.J.Moore,S.P.Ong,C.C.Fischer,T.Mueller,K.A.Persson,G.Ceder,A high-throughput infrastructure for density functional theory calcula-tions,Computation Materials Science50(2011)2295.[10]S.P.Ong,V.L.Chevrier,G.Ceder,Comparison of small polaron migration andphase separation in olivine LiMnPO4and LiFePO4using hybrid density func-tional theory,Physical Review B83(075112)(2011)1.[11]G.Ceder,Opportunities and challenges forfirst-principles materials design andapplications to Li battery materials,MRS Bulletin35(2010)693.[12]X.Zhang,Multiscale Modeling of Li-ion Cells:Mechanics,Heat Generation andElectrochemical Kinetics,University of Michigan,2009(Ph.D.thesis).[13]A.M.Colclasure,K.A.Smith,R.J.Kee,Modeling detailed chemistry and transportfor solid-electrolyte-interface(SEI)films in Li-ion batteries,Electrochimica Acta 58(2011)33.[14]tz,J.Zausch,Thermodynamic consistent transport theory of Li-ion batter-ies,Journal of Power Sources196(2011)3296.[15]O.V.Bushkova,O.L.Andreev,N.N.Batalov,S.N.Shkerin,M.V.Kuznetsov,A.P.Tyutyunnik,O.V.Koryakova,E.H.Song,H.J.Chung,Chemical interactions in the cathode half-cell of lithium-ion batteries:Part I.Thermodynamic simulation, Journal of Power Sources157(2006)477.[16]H.Yokokawa,N.Sakai,K.Yamaji,T.Horita,M.Ishikawa,Thermodynamic deter-mining factors of the positive electrode potential of lithium batteries,Solid State Ionics113–115(1998)1.[17]A.Van der Ven,G.Ceder,First principles calculation of the interdiffusion coef-ficient in binary alloys,Physical Review Letters94(2005)1.[18]A.Van der Ven,G.Ceder,M.Asta,P.D.Tepesch,First-principles theory of ionicdiffusion with nondilute carriers,Physical Review B64(2001)1.[19]M.V.Koudriachova,N.M.Harrison,S.W.de Leeuw,First principles predictionsfor intercalation behaviour,Solid State Ionics175(2004)829.[20]A.Van der Ven,G.Ceder,Lithium diffusion in layered Li X CoO2,Electrochemicaland Solid-State Letters3(2000)301.[21]D.Morgan,A.Van der Ven,G.Ceder,Li conductivity in Li X MPO4M=(Mn,Fe,Co,Ni)olivine materials,Electrochemical and Solid-State Letters7(2004)A30.[22]M.K.Aydinol,A.F.Kohan,G.Ceder,K.Cho,J.Joannopoulos,Ab initio studyof lithium intercalation in metal oxides and metal dichalcogenides,Physical Review B56(1997)1354.[23]T.-F.Yi,Y.-R.Zhu,R.-S.Zhu,Density functional theory study of lithium inter-calation for5V LiNi0.5Mn1.5O4cathode materials,Solid State Ionics179(2008) 2132.[24]Ma Xi,B.Kang,G.Ceder,High rate micron-sized ordered LiNi0.5Mn1.5O4,Journalof the Electrochemical Society157(2010)A925.[25]X.Fang,N.Ding,X.Y.Feng,Y.Lu,C.H.Chen,Study of LiNi0.5Mn1.5O4syn-thesized via a chloride-ammonia co-precipitation method:electrochemical performance,diffusion coefficient and capacity loss mechanism,Electrochim-ica Acta54(2009)7471.[26]M.Mohamedi,M.Makino,K.Dokko,T.Itoh,I.Uchida,Electrochemical investi-gation of LiNi0.5Mn1.5O4thinfilm intercalation electrodes,Electrochimica Acta 48(2002)79.[27]/∼mdt26/casino2pseudopotentials.html(accessed01.10.13).[28]V.I.Anisimov,J.Zaanen,O.K.Andesen,Band theory and Mott insulators:Hub-bard U instead of Stoner I,Physical Review B44(1991)493.[29]L.Wang,Th.Maxisch,G.Ceder,Oxidation energies of transition metal oxideswithin the GGA+U framework,Physical Review B73(2006)1.[30]D.Kramer,G.Ceder,Tailoring the morphology of LiCoO2:afirst principles study,Chemistry of Materials21(2009)3799.[31]A.Van der Ven,First Principle Investigation of the Thermodynamicand Kinetic Properties of Lithium Transition Metal Oxides,MIT,2000 (Ph.D.thesis).[32]M.Strickland, D.Yager-Elorriaga,A parallel algorithm for solving the3d Schrodinger equation,Journal of Computational Physics229(2010) 6015.。
electrochimica acta参考文献格式
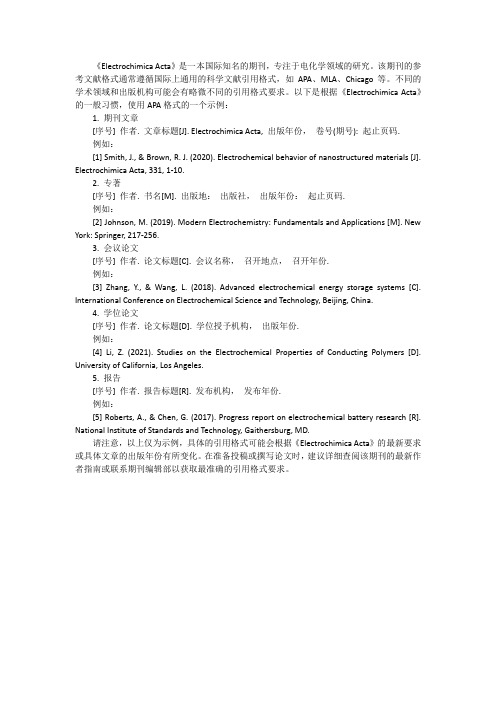
《Electrochimica Acta》是一本国际知名的期刊,专注于电化学领域的研究。
该期刊的参考文献格式通常遵循国际上通用的科学文献引用格式,如APA、MLA、Chicago等。
不同的学术领域和出版机构可能会有略微不同的引用格式要求。
以下是根据《Electrochimica Acta》的一般习惯,使用APA格式的一个示例:1. 期刊文章[序号] 作者. 文章标题[J]. Electrochimica Acta, 出版年份,卷号(期号): 起止页码.例如:[1] Smith, J., & Brown, R. J. (2020). Electrochemical behavior of nanostructured materials [J]. Electrochimica Acta, 331, 1-10.2. 专著[序号] 作者. 书名[M]. 出版地:出版社,出版年份:起止页码.例如:[2] Johnson, M. (2019). Modern Electrochemistry: Fundamentals and Applications [M]. New York: Springer, 217-256.3. 会议论文[序号] 作者. 论文标题[C]. 会议名称,召开地点,召开年份.例如:[3] Zhang, Y., & Wang, L. (2018). Advanced electrochemical energy storage systems [C]. International Conference on Electrochemical Science and Technology, Beijing, China.4. 学位论文[序号] 作者. 论文标题[D]. 学位授予机构,出版年份.例如:[4] Li, Z. (2021). Studies on the Electrochemical Properties of Conducting Polymers [D]. University of California, Los Angeles.5. 报告[序号] 作者. 报告标题[R]. 发布机构,发布年份.例如:[5] Roberts, A., & Chen, G. (2017). Progress report on electrochemical battery research [R]. National Institute of Standards and Technology, Gaithersburg, MD.请注意,以上仅为示例,具体的引用格式可能会根据《Electrochimica Acta》的最新要求或具体文章的出版年份有所变化。
ew approach for synthesis of carbon-mixed LiFePO4 cathode materials

Electrochimica Acta50(2004)421–426New approach for synthesis of carbon-mixed LiFePO4cathode materialsK.Konstantinov a,∗,S.Bewlay a,G.X.Wang a,M.Lindsay a,J.Z.Wang a,H.K.Liu a,S.X.Dou a,J.-H.Ahn ba Institute for Superconducting and Electronic Materials,University of Wollongong,Northfields Ave.,2522Wollongong,NSW,Australiab Department of Materials Engineering,Andong National University,388Songchun-Dong,760-749Andong,Gyungbuk,South KoreaReceived2June2003;received in revised form10May2004;accepted10May2004Available online30July2004AbstractFor thefirst time,carbon-mixed LiFePO4(LFP)cathode materials have been prepared by spray solution technology.Nominal addition of 15or20wt.%C was used in order to simulate the industrial practice for preparation of electrode materials.The prepared powders consist of a single LiFePO4phase:small crystallites with a highly developed surface area,beneficial for the surface electrochemical processes limited by the low Li diffusion.The combination of spray technology and carbon addition increased the specific surface area above20m2/g at a relatively high sintering temperature(700◦C).The initial discharge capacity was up to140mAh/g compared to125mAh/g for conventionally prepared (using a solid state reaction)LiFePO4electrode materials.©2004Elsevier Ltd.All rights reserved.Keywords:Carbon-mixed LiFePO4;Cathode materials;Spray solution1.IntroductionThe continuously increasing demand for higher per-formance and cheaper rechargeable batteries for different electronic devices during the last decade has forced re-searchers to study new classes of materials for replacement of the conventional Li-ion and NiMH materials.Among the various materials suitable for use as cathodes in Li-ion batteries,the phospho-olivine family and especially the LiFePO4(LFP)member has recently attracted significant interest because of its low cost,low hygroscopicity and environmentally friendly components.In different papers, authors have reported improved synthesis methods,which aim to overcome the main disadvantages of LiFePO4, namely its poor conductivity and long sintering procedures [1–5].In a number of papers,preparation of carbon-included LFP has been described and studied[6–8]in order to use the reduction properties of carbon and avoid formation of ∗Corresponding author.Tel.:+61242215765;fax:+61242215731.E-mail address:konstan@.au(K.Konstantinov).the Fe3+oxidation state,as well as to increase the electronic conductivity of these materials.Such papers emphasized the positive influence of C,but generally they do not aim to prepare thefinal electrode mixture used in the industry (where the active cathode material is usually mixed with 10–15%carbon black in order to increase conductivity).In this work,we report for thefirst time the production of homogeneous carbon/LiFePO4electrode-ready powders bya spray solution method.2.Experimental proceduresAs an electronic-conducting precursor,different amounts of sucrose(C12H22O11)were added to a water-based HNO3 solution of lithium carbonate,iron oxalate and ammonium di-hydrogen phosphate containing Li:Fe:P in the stoichio-metric ratio=1:1:1.The sucrose solution was added to en-sure nominal carbon content15or20wt.%in the mixture. These values are close to the amount of carbon black mixed with electrode material in the battery industry.The solution0013-4686/$–see front matter©2004Elsevier Ltd.All rights reserved. doi:10.1016/j.electacta.2004.05.049422K.Konstantinov et al./Electrochimica Acta 50(2004)421–426was sprayed in a heat reactor at 450◦C and the obtained pre-cursor was briefly sintered at 700◦C (for 4h).This regime was found to be most appropriate to produce the finest pow-der and therefore to promote Li diffusion,which is a limit-ing factor in LFP materials.In some cases,the powder was slightly compacted before the final sintering.The materials were analyzed by X-ray diffraction analysis (XRD),scan-ning electron microscopy (SEM)and a gas sorption technique to evaluate the specific surface area by the BET method.For electrochemical characterization,Li-ion cells were as-sembled in which the carbon-containing LFP materials were used as a cathode material and graphite powder was used as an anode material.One mole LiPF 6in a 1:1mixture with ethylene carbonate and dimethyl carbonate was used as the electrolyte.3.Results and discussionThe results from the XRD analysis show that the obtained materials consist of a single LiFePO 4phase right throughout the concentration range of added carbon (Fig.1).The car-bon is in amorphous form,as there are no additional peaks on the XRD patterns belonging to its crystal modification.The SEM microstructural observations (Fig.2)reveal the strong effect of carbon on the microstructure of LFP pow-ders.Highly developed surface area and spherical agglom-erates are observed (Fig.2b and c).The carbon-free LFP powder (Fig.2a)prepared via solid-state reaction method re-vealed much larger grains and dense structure.The increase of carbon addition at 20leads to appearance of more hollow particles,“donut”structures and other deviations from the spherical shape (Fig.2d).Fig.1.XRD patterns of pure LiFePO 4material (a),15wt.%carbon mixed LiFePO 4powder obtained by spray solution method (b)and 20wt.%carbon mixed LiFePO 4powder obtained by spray solution method (c).To evaluate quantitatively the carbon influence on the mi-crostructure,BET analysis was performed and the results are shown in Table 1.From these results is obvious that the car-bon addition leads to highly developed surface area,which exceeds 20m 2/g.Such values according to [10]can be ob-tained at only 400◦C sintering temperature if a classical solid-state reaction method is applied.The spray solution method used by us produces a much higher specific surface area for pure LFP (around 5m 2/g)compared to samples prepared by solid-state reaction method (less than 1m 2/g).This is due to the almost instant chemical decomposition and short reaction time used.The resistivity measurements shown in Table 2demon-strate the significant increase of conductivity for carbon-included LFP powders.The improvement is around four or-ders for 20wt.%carbon inclusion.The improved conduc-tivity is due to the homogeneous mixture of LFP grains in amorphous carbon,which decreases the resistivity at grain boundaries of active material.The improved conductivity is expected to have a positive effect on the capacity of batteries.The electrochemical properties and the influence of the carbon concentration on the battery performance were also investigated.Fig.3shows the initial charge/discharge cycles of LFP powder with 15%C addition.This material demon-strated the highest discharge capacity value of 140mAh/g,whereas the 20%C addition LFP powder achieved only 125mAh/g.This may be due to the lower mass of LFP ac-tive material compared to powder with 15%nominal carbon addition.The result indicates that 15%carbon addition is the optimum level,which helps LFP to reach closer to the theoretical maximum capacity of 170mAh/g.The typical in-cremental capacities versus voltage obtained for carbon/LFP materials are shown in Fig.4.The large charge/dischargeK.Konstantinov et al./Electrochimica Acta50(2004)421–426423 Table1Specific surface area of LFP/carbon mixed powders prepared by spray solution methodSample number Remarks Carbon content(wt.%)Specific surface area determined bymultipoint BET method(m2/g)1Reference sample00.882Spray pyrolysis05.983Spray pyrolysis1512.194Spray pyrolysis2021.28The reference sample was prepared by classical solid-state reaction method decomposed at350◦C for10h,reground and sintered in Ar for22h.Fig.2.SEM images of LFP materials prepared via solid-state reaction method(a),spray solution method with15wt.%carbon(b),and different magnifications of LFP powder with20wt.%carbon obtained by spray solution method(c and d).424K.Konstantinov et al./Electrochimica Acta50(2004)421–426Fig.2.(Continued).K.Konstantinov et al./Electrochimica Acta 50(2004)421–426425Table 2Conductivity of carbon-included LFP materials prepared by spray solution methodNominal carbon addition (wt.%)Conductivity (S/cm 2)0 4.6×10−815 3.6×10−5202.4×10−4Fig.3.Typical charge/discharge vs.capacity cycles of 15wt.%carbon added LFP by spray pyrolysis.First charge/discharge cycle (—);second charge/discharge cycle (---).The charge/discharge current is 0.02mA/g.The cycling potential range is 3.0–4.4V.Fig.4.Typical incremental capacity/voltage dependencies of 20wt.%LFP/carbon mixed material prepared via spray solution method.peaks also indicate the fine microstructure of the materials.The charge peak is positioned at 3.49V and the discharge one at 3.38V .4.ConclusionsUsing a spray solution technique,electrode-ready LiFePO 4cathode powders with nominal carbon addition 15or 20wt.%were successfully prepared.The materials are sin-gle LFP phase and feature much finer microstructure and highly developed surface area,compared to materials pre-pared by classical solid-state reaction methods.The first dis-charge cycle capacity is up to 140mAh/g.The conductivity of carbon-added LFP powders was four orders of magnitude higher than carbon-free LFP material.AcknowledgementsThis work has been supported financially by the Australian Research Council,Sons of Gwalia Ltd.,OM Group Inc.and Lexel Battery (Shenzhen)Co.Ltd.References[1]F.Croce, A.D.Epifanio,J.Hassoun, A.Deptula,T.Olczac, B.Scrosati,Electrochem.Solid State Lett.5(2002)47.[2]P.P.Prosini,M.Carevska,S.Scaccia,P.Wisniewski,S.Passerini,M.Pasquali,J.Electrochem.Soc.149(2002)A886.426K.Konstantinov et al./Electrochimica Acta50(2004)421–426[3]A.Yamada,S.C.Chung,K.Hinokuma,J.Electrochem.Soc.148(2001)A224.[4]M.Takahashi,S.Tobishima,K.Takei,Y.Sakurai,J.Power Sources9798(2001)508.[5]S.-Y.Chung,J.T.Bloking,Y.-M.Chiang,Nat.Mater.1(2002)123.[6]J.Baker,M.Y.Saidi,J.L.Swoyer,Electrochem.Solid State Lett.6(2003)A53.[7]S.Franger,F.Le Cras,C.Bourbon,H.Rouault,Electrochem.SolidState Lett.5(2002)A231.[8]Z.Chen,J.R.Dahn,J.Electrochem.Soc.149(2002)A1184.。
Electrochemical processes in macro and microfluidic cells for the abatement of chloroacetic acid
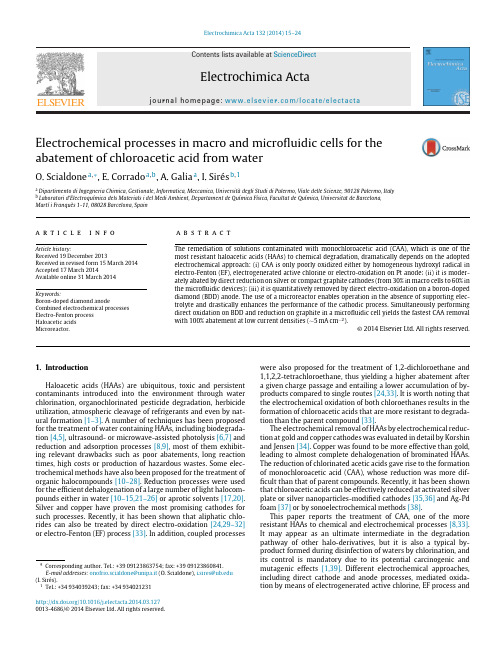
Electrochimica Acta 132(2014)15–24Contents lists available at ScienceDirectElectrochimicaActaj o u r n a l h o m e p a g e :w w w.e l s e v i e r.c o m /l o c a t e /e l e c t a c taElectrochemical processes in macro and microfluidic cells for the abatement of chloroacetic acid from waterO.Scialdone a ,∗,E.Corrado a ,b ,A.Galia a ,I.Sirés b ,1aDipartimento di Ingegneria Chimica,Gestionale,Informatica,Meccanica,Universitàdegli Studi di Palermo,Viale delle Scienze,90128Palermo,Italy bLaboratori d’Electroquímica dels Materials i del Medi Ambient,Departament de Química Física,Facultat de Química,Universitat de Barcelona,Martíi Franquès 1-11,08028Barcelona,Spaina r t i c l ei n f oArticle history:Received 19December 2013Received in revised form 15March 2014Accepted 17March 2014Available online 31March 2014Keywords:Boron-doped diamond anodeCombined electrochemical processes Electro-Fenton process Haloacetic acids Microreactor.a b s t r a c tThe remediation of solutions contaminated with monochloroacetic acid (CAA),which is one of the most resistant haloacetic acids (HAAs)to chemical degradation,dramatically depends on the adopted electrochemical approach:(i)CAA is only poorly oxidized either by homogeneous hydroxyl radical in electro-Fenton (EF),electrogenerated active chlorine or electro-oxidation on Pt anode;(ii)it is moder-ately abated by direct reduction on silver or compact graphite cathodes (from 30%in macro cells to 60%in the microfluidic devices);(iii)it is quantitatively removed by direct electro-oxidation on a boron-doped diamond (BDD)anode.The use of a microreactor enables operation in the absence of supporting elec-trolyte and drastically enhances the performance of the cathodic process.Simultaneously performing direct oxidation on BDD and reduction on graphite in a microfluidic cell yields the fastest CAA removal with 100%abatement at low current densities (∼5mA cm −2).©2014Elsevier Ltd.All rights reserved.1.IntroductionHaloacetic acids (HAAs)are ubiquitous,toxic and persistent contaminants introduced into the environment through water chlorination,organochlorinated pesticide degradation,herbicide utilization,atmospheric cleavage of refrigerants and even by nat-ural formation [1–3].A number of techniques has been proposed for the treatment of water containing HAAs,including biodegrada-tion [4,5],ultrasound-or microwave-assisted photolysis [6,7]and reduction and adsorption processes [8,9],most of them exhibit-ing relevant drawbacks such as poor abatements,long reaction times,high costs or production of hazardous wastes.Some elec-trochemical methods have also been proposed for the treatment of organic halocompounds [10–28].Reduction processes were used for the efficient dehalogenation of a large number of light halocom-pounds either in water [10–15,21–26]or aprotic solvents [17,20].Silver and copper have proven the most promising cathodes for such processes.Recently,it has been shown that aliphatic chlo-rides can also be treated by direct electro-oxidation [24,29–32]or electro-Fenton (EF)process [33].In addition,coupled processes∗Corresponding author.Tel.:+3909123863754;fax:+3909123860841.E-mail addresses:onofrio.scialdone@unipa.it (O.Scialdone),i.sires@ (I.Sirés).1Tel.:+34934039243;fax:+34934021231were also proposed for the treatment of 1,2-dichloroethane and 1,1,2,2-tetrachloroethane,thus yielding a higher abatement after a given charge passage and entailing a lower accumulation of by-products compared to single routes [24,33].It is worth noting that the electrochemical oxidation of both chloroethanes results in the formation of chloroacetic acids that are more resistant to degrada-tion than the parent compound [33].The electrochemical removal of HAAs by electrochemical reduc-tion at gold and copper cathodes was evaluated in detail by Korshin and Jensen [34].Copper was found to be more effective than gold,leading to almost complete dehalogenation of brominated HAAs.The reduction of chlorinated acetic acids gave rise to the formation of monochloroacetic acid (CAA),whose reduction was more dif-ficult than that of parent compounds.Recently,it has been shown that chloroacetic acids can be effectively reduced at activated silver plate or silver nanoparticles-modified cathodes [35,36]and Ag-Pd foam [37]or by sonoelectrochemical methods [38].This paper reports the treatment of CAA,one of the more resistant HAAs to chemical and electrochemical processes [8,33].It may appear as an ultimate intermediate in the degradation pathway of other halo-derivatives,but it is also a typical by-product formed during disinfection of waters by chlorination,and its control is mandatory due to its potential carcinogenic and mutagenic effects [1,39].Different electrochemical approaches,including direct cathode and anode processes,mediated oxida-tion by means of electrogenerated active chlorine,EF process and/10.1016/j.electacta.2014.03.1270013-4686/©2014Elsevier Ltd.All rights reserved.16O.Scialdone et al./Electrochimica Acta132(2014)15–24coupled systems have been investigated in order to compare the performance of each route andfinally propose the most suitable one.One of the main drawbacks that are inherent to the electro-chemical technologies operated in conventional cells arises from the need of a high conductivity in order to minimize the ohmic drop in the aqueous solution and the overall potential difference between the anode and cathode.Consequently,the addition of a certain amount of supporting electrolyte is usually a must.This is certainly an important obstacle for the widespread introduc-tion of many electrochemical technologies in the market,as in the case of water decontamination.Indeed,the addition of chemicals to polluted water streams is often a problematic issue,being con-trary to general administrative regulations because this may lead to the formation of secondary pollutants during the electrochemical degradation of initial contaminants.Furthermore,it increases the operation costs.Recently,different research groups have demon-strated the successful application of microfluidic electrochemical reactors(i.e.,cells with a distance of tens or hundreds of microme-ters between the anode and cathode)to both,the electrochemical treatment of aqueous solutions of organic pollutants[40–44]and the synthesis offine chemicals[45–48],in the absence of suppor-ting electrolytes with a small potential difference between both electrodes.Therefore,the drastic reduction of distances between electrodes leads to a major reduction of the ohmic resistance. Furthermore,higher current efficiencies were achieved in microre-actors for the electrochemical abatement of organic pollutants performed by both direct anodic oxidation and EF processes caused by an intensification of mass transport phenomena[41–44].Hence, the treatment of aqueous solutions of CAA has been carried out in macro and microfluidic devices equipped with different anode and cathode materials in order to improve the performance of the elec-trochemical processes under study in terms of CAA and chemical oxygen demand(COD)abatement.2.ExperimentalTwo macrofluidic cells,namely system I and system II,and a microfluidic cell were used to carry out galvanostatic electroly-ses by employing an Amel2053potentiostat-galvanostat and a 3300current integrator.Macro-scale electrolyses were performed in batch mode.System I was a bench,open,undivided,cylindrical glass cell of150mL capacity with a double jacket for circulation of external thermostated water to regulate the solution tempera-ture at25◦C.The anode was either a3cm2Pt sheet(SEMPSA)or a3cm2BDD thin-film electrode(Condias).The cathode was a3 cm2carbon-PTFE air-diffusion electrode(ADE)from E-TEK,which was fed with air at1.0L min−1for ensuring continuous H2O2 electrogeneration from the two-electron reduction of O2.The inter-electrode gap was about1cm.The solutions were always vigorously stirred with a magnetic bar at800rpm to enable mixing and trans-port of parative experiments were performed with 100mL of solution.For the photoelectro-Fenton(PEF)process,a Philips TL/6W/08fluorescent black light blue tube(320-400nm withmax=360nm)was placed above the solution.System II was equipped with an undividedfilter-press reactor(ElectroCell AB)to work in aflow configuration.This reactor was composed of a BDD (Condias)or Ti/IrO2-Ta2O5(ElectroCell AB)anode and a cathode made of either compact graphite(Carbone Lorraine),Ag(Electro-Cell AB),Cu or AISI304stainless steel plate.The exposed surface area of the plates was10cm2and the interelectrode gap was4mm. Comparative experiments were performed with200or300mL of solutions,which were placed in a jacketed glass reservoir of350mL and sparged with nitrogen or compressed air.The solutions were continuously recirculated at1L min−1by a centrifugal pump.The experiments in the microreactor were performed inflow mode with a single passage of the solution through the cell,which was enabled by a syringe pump(New Era Pump Systems,Inc.)that fed the solutions at aflow rate between0.05and0.6mL min−1.The microreactor consisted in the abovementioned commercial undi-videdfilter-pressflow cell from ElectroCell AB,now equipped with one or two PTFE spacers(thickness of the spacer in the microme-ter range,i.e.,50or100m)placed between the electrodes,which were selected among those used in system II(see references[41,48] for a more detailed description of the micro device).Thus,BDD or Ti/IrO2-Ta2O5and compact graphite or stainless steel,were employed as anode and cathode,respectively.The spacers were cut so as to expose a working area of5cm2.For each tested operating condition,at least2samples of1mL were analyzed.The concentrations of CAA and acetic acid were evaluated by high-performance liquid chromatography(HPLC)using an HP1100 LCfitted with a Prevail Organic Acid5column(Grace Davison Dis-covery Sciences)at25◦C and coupled with a UV detector selected at =210nm.A25mM KH2PO4aqueous solution at pH2.0(H3PO4) was eluted at1.0mL min−1as the mobile phase.The concentra-tion of accumulated H2O2was determined spectrophotometrically from the light absorption of the Ti(IV)–H2O2colored complex at =409nm,by using titanium(IV)oxysulfate(TiOSO4·H2O)from Fluka.The COD was determined by using commercial Merck cell tests.The total organic carbon(TOC)abatement was evaluated by using a Shimadzu VCSN TOC analyzer.The oxychlorine ions(chlo-rate and perchlorate)were quantified by ion chromatography(IC) using a Shimadzu10Avp HPLCfitted with a Shim-Pack IC-A1S (100mm×4.6mm(i.d.))anion column at40◦C and coupled with a Shimadzu CDD10Avp conductivity detector.The mobile phase was a solution with2.4mM tris(hydroxymethyl)aminomethane and2.5mM phthalic acid at pH4.0eluted at1.0mL min−1.All the species were identified by comparison of their retention times with those of pure standards.The electrolyses were performed using bidistilled water to prepare solutions of5mM CAA(Merck),which usually contained 0.035M Na2SO4(Janssen Chimica)as supporting electrolyte for macro-scale experiments.For the study of electrochemical Fenton processes,0.25-1.0mM FeSO4(Fluka)was added as catalyst.Sulfu-ric acid(Aldrich)was used to adjust the pH of the solution when necessary.Prior to each experiment with the BDD anodes,an anodic polarization was carried out at3.0V vs.SCE for5min.In the case of silver,the surface was polished with alumina powder and then activated by means of either an oxidation–reduction cycle process, at10mV s−1in the range from-0.4to0.4V(4cycles,at298K)or amperostatic pulses in Na2SO4or pact graphite and cop-per cathodes were mechanically polished with alumina powder.The percentage of abatement(i.e.,the CAA conversion and the COD removal)was defined by Eq.(1),while the current efficiency (CE,%)for the conversion of the pollutant was defined by Eqs.(2) and(3)for macro and a microfluidic cells,respectively.X=100C0−C fC0(1) CE=100nFVC0Xapp(2) CE=100nFC0ϕV Xi app A(3)where C0and C f are the initial andfinal CAA concentrations or COD values,n is the number of required electrons for the conversion of the pollutant to carbon dioxide for oxidation processes(6)and to acetic acid for the reduction route(2),F the Faraday constant (96487C mol−1),i app the applied current density,A the exposedO.Scialdone et al./Electrochimica Acta132(2014)15–2417 Table1Abatement of CAA by various electrochemical routes.aEntry Process System(reactor)Current density(A m−2)Flow rate(mL min−1)Time(h)b Abatement(%)and(Current efficiency(%))1c EF or PEF with an ADE and electro-oxidation on Pt System I1000>5Not significant2Cathodic reduction on System II•copper4005<10(<0.5)•silver d400527(1.1)•graphite e100528(4.4)(Anode:Ti/IrO2-Ta2O5)3Electro-oxidation on BDD System II100589(42) (Cathode:stainless steel)4Electro-oxidation on BDD and cathodic reductionon graphiteSystem II100591(43)f5e Cathodic reduction on graphite System III(micro)1000.4 6.340(5.1) (Anode:Ti/IrO2-Ta2O5)800.5536(7.2)6g Electro-oxidation on BDD System III(micro)2000.38100(15) (Cathode:stainless steel)1000.5587(42)500.3875(44)7e Electro-oxidation on BDD and cathodic reductionon graphiteSystem III(micro)2000.38100(15)f1000.5595(46)f500.3886(50)fa Electrolyses of aqueous solutions of5mM CAA,using Na2SO4as supporting electrolyte for conventional reactors I and II.Unless stated otherwise,the considered volume was300mL.b Normalized time for the treatment of300mL of solutions considering a nominal geometric electrode surface of10cm2.Actually,system II has an electrode surface of10 cm2.In the case of the microreactor,the calculation is made by considering the utilization of two microreactors(each one with an electrode surface of5cm2)in parallel.c100mL of aqueous solution(pH3.0).d Preliminary activation of the silver cathode by two oxidation(10mA cm−2)and reduction(-10mA cm−2)amperostatic pulses(2min each).e At pH2.7,in the presence of0.5mM FeSO4.Nominal distance between the electrodes:100m.f Current efficiency conventionally calculated by considering the oxidation of CAA to CO2.g Nominal distance between the electrodes:50m.area,t the average residence time of the solution in the cell,V the solution volume and V the volumetricflow rate.Note that,if gas bubbles are formed at the electrodes,their presence can affect the mass transport of the organic molecules toward/from the electrode surface.Furthermore,the gas evolu-tion can result in a decrease of the effective surface area.However, according to the literature,for macro cells there exists an extended current density region(up to about100–1000A m−2)in which the convection caused by gas bubbles is not strong enough so as to modify the mass transport mechanism[57].Gas evolution can also affect dramatically theflow pattern in microreactors,although it did not seem to exert a remarkable influence on the abatement of organic pollutants for current densities up to about23mA cm−2, when the process was controlled by the mass transport of the pol-lutant toward the anode surface[41].At higher current densities, the mass transport rate of the pollutant(and,as a consequence, its abatement)was enhanced.Most of the trials reported in the present work were carried out with quite low current densities (<25mA cm−2).Nevertheless,the partial coverage of the elec-trode surface by gas bubbles cannot be discarded and hence,all the current densities are the nominal ones since they refer to the ratio between the applied current and the geometric electrode surface.3.Results and discussion3.1.Electrolyses in conventional cellsAfirst series of experiments was performed in conventional macrofluidic cells to assess the effect of the electrochemical route on the removal of CAA from contaminated,synthetic aqueous solutions.The performances of reduction processes on silver,cop-per and graphite cathodes,direct electro-oxidation on Pt and BDD,mediated oxidation by electrogenerated active chlorine,EF and PEF processes and some coupled processes were carefully investigated.3.1.1.EF and PEF processesIn EF process,oxygen is usually reduced at carbonaceous cathodes to hydrogen peroxide(Eq.(4))that,in the presence of catalytic amounts of iron(II),is converted to hydroxyl radicals(Eq.(5))that can oxidize organic pollutants in aqueous solution[49]. In undivided cells,which are preferred to avoid the high voltage penalty caused by separators,the required O2is directly fed as compressed air through an ADE or can be simply provided by the oxidation of water at the anode(Eq.(6))when graphite or carbon felt are used as cathode materials.Cathode)O2+2H++2e−→H2O2(4) Fe2++H2O2→Fe3++•OH+OH−(5) Anode)H2O→0.5O2+2H++2e−(6) Preliminary experiments were carried out by using system I as the macro cell,aiming to evaluate the production of hydrogen per-oxide in an undivided cell equipped with an ADE cathode and a Pt anode.A steady concentration of H2O2of about50mM was achieved after6h at300mA,pH3.0and25◦C with0.035M Na2SO4 as supporting electrolyte.When experiments were repeated in the presence of5mM CAA,with or without addition of0.5mM FeSO4, no significant abatements of either CAA or TOC were observed after protracted electrolyses,thus demonstrating that neither electro-oxidation on Pt nor EF process are able to degrade this resistant molecule(Table1,entry1).CAA was also resistant to PEF process performed with UVA light irradiation under the same operat-ing conditions.Quite interestingly,when the electrolyses were repeated on compact graphite cathode,the TOC still remained unchanged but a significant conversion of CAA to acetic acid was observed,presumably as a result of a reduction process(see the following section).18O.Scialdone et al./Electrochimica Acta132(2014)15–24Fig.1.Effect of pre-treatment of silver cathode on the cathode abatement of chloroacetic acid(CAA).The electrolyses of300mL of solution were carried out in system II at40mA cm−2in the same medium used for the pre-treatment stage after the addition of5mM CAA.Anode:Ti/IrO2-Ta2O5.Electrode surface:10cm2. Pre-treatment:( )Two oxidation(1mA cm−2)and reduction(-1mA cm−2)amper-ostatic pulses(2min each)in0.035M Na2SO4aqueous solution;two oxidation (10mA cm−2)and reduction(-10mA cm−2)amperostatic pulses(2min each)in:(᭹) 0.035M Na2SO4,( )0.5M Na2SO4or( )0.5M NaCl;( )four oxidation–reduction cycles in0.035M Na2SO4(scan rate of10mV s−1ranging from-0.4to0.4V).3.1.2.Electroreduction processThe galvanostatic reduction of CCA was carried out using system II on silver and copper cathodes,the more promising materials, according to literature,for the cathode reduction of chlorinated compounds and,in particular,of HAAs[34–37].Based on the above mentioned results,compact graphite was tested as well.An iridium-based electrode was used as the anode to favor the oxygen evolution reaction(Eq.(6)).The expected reduction process at the cathode was:ClCH2COOH+H++2e−→CH3COOH+Cl−(7) First experiments carried out at different current densities with mechanically polished silver gave very low CAA abatements(<7%) even after5h.Xu and co-authors have shown that the cathode abatement of HAAs on silver can be enhanced if the electrode is pretreated using an oxidation–reduction cycling method[35].Also in our case such a pretreatment enhanced the CAA abatements.As shown in Fig.1,similar trends(ending in ca.12%CAA abatement at 5h)were achieved activating the electrode in an aqueous solution of0.035M Na2SO4by both a cyclic voltammetry procedure(four oxidation–reduction cycles,scan rate of10mV s−1in the range from -0.4to0.4V vs.SCE similar to that proposed by Xu et al.[35])or by means of two oxidation and reduction amperostatic pulses(10and -10mA cm−2).A lower CAA abatement of about7%was obtained using the galvanostatic pulse pretreatment at lower current den-sity,whereas a higher degradation of about27%at5h was achieved upon increase of the supporting electrolyte concentration to0.5M (Fig.1).The use of NaCl instead of Na2SO4did not lead to any signif-icant change.In all cases,the abatement of CAA led to the formation of corresponding amounts of acetic acid.However,a rather low CAA abatement(<30%)was achieved in all experiments despite the high charge passed,therefore resulting in very low current efficiencies(< 2%).When experiments were repeated at a copper cathode,signif-icantly lower abatements were achieved(<10%after5h at20and 40mA cm−2).A quite complex picture was observed on graphite cathodes.In particular,it was observed that this material gave rise to a reduction of CAA to acetic acid while the solution TOC remained unchanged.Worth noting,the addition of small amounts of iron(II) at pH2.7allowed reaching even higher conversions of CAA to acetic acid.As shown in Fig.2,the performance of the reduction process with this cathode strongly depended on the current density and solution pH.A higher CAA abatement,always<30%,was achieved at an intermediate current density value of10mA cm−2and at a higher pH value,as shown in Fig.2A and2B,respectively.In order to understand the role of iron ions and the effect of pH,one can con-sider that p K a of CAA is2.87[50].Hence,at the employed pH values, both the dissociated and non-dissociated forms of CAA are present in solution.Chloroacetate ion(ClCH2COO−),whose concentration increases with pH,is expected to become complexed by iron(III) formed in the medium,thus appearing as a cationic species such as[Fe(OOCCH2Cl)]2+[51],which is likely to be more easily reduced than both ClCH2COO−and ClCH2COOH.When the reduction exper-iments were repeated on silver cathode,quite similar results were achieved both in the absence and presence of iron(II).Hence,the effect of iron ions is likely to be due to specific interactions with the graphite surface.As shown in Fig.2B(see image(a)as an example),at the end of the electrolyses performed with graphite at higher pH(i.e.,2.5 and2.7)some iron deposits appeared on the edge of the cathode plate,where the mixing of solution is expected to be less effec-tive.The iron precipitation is expected to take place at pH values higher than3.Hence,the phenomenon was probably caused by a local increase of pH due to the parallel hydrogen evolution reaction (Eq.(8))in the area where the mixing is less effective.As shown in Fig.2B,the utilization of pH close to2prevented the precipitation on the cathode surface but gave rise to lower abatements.In con-clusion,silver and graphite cathodes can be used to reduce CAA but, in both cases,under best adopted operating conditions rather low abatements close to30%were achieved in macro cells.H2O+e−→OH−+0.5H2(8) 3.1.3.Direct electro-oxidation processThe direct electro-oxidation of CAA was carried out on Pt and BDD,which according to literature is the most promising material for the anodic oxidation of aliphatic organochlorinated compounds [31].Stainless steel was used as an inert cathode to favor the hydro-gen evolution.The main expected redox processes are then given by Eq.(9)on the anode and Eq.(8)on the cathode.ClCH2COOH+2H2O→2CO2+Cl−+7H++6e−(9) While on Pt no CAA abatement occurred,on BDD the direct oxi-dation process allowed a very fast and complete degradation of CAA,as shown in Fig.3.The trends of both CAA and TOC abate-ments were very similar,thus showing that electro-oxidation at BDD allows the effective conversion of CAA to carbon dioxide.An increase of current density(i)from5to40mA cm−2resulted in a faster abatement of CAA(Fig.3,curves a-d)but in a progressively lower current efficiency(CE=36%and20%at20and40mA cm−2, respectively,after3h).Indeed,at higher current densities the pro-cess is likely to be under mass transport control(i.e.,transport of CAA towards the anodic surface)or under a mixed control regime and,consequently,a larger fraction of current density is used for parasitic processes such as the oxygen evolution.It has been reported in literature that chloride ions can be oxi-dized on BDD to form chlorate and perchlorate[33,52,53].As shown in Fig.4A,at40mA cm−2,the abatement of CAA led to the forma-tion of corresponding amounts of both oxychlorine ions,although they presented different profiles.In particular,the curve for chlo-rate concentration vs.time exhibited a maximum,which can be explained by the conversion of chlorides to chlorate and the subse-quent transformation of chlorate to perchlorate under the action of hydroxyl radicals formed on BDD.At the end of the electrolysis,the oxychlorine ions were mainly accumulated in the form of perchlo-rate.The concentration trends at100mA cm−2can be commented in the same manner,as depicted in Fig.4B and4C,although a faster decay of chlorate was accompanied by a quicker accumulation ofO.Scialdone et al./Electrochimica Acta132(2014)15–2419Fig.2.Effect of(A)current density and(B)pH on the abatement of CAA using compact graphite as cathode.Plot A:( )5,( )10and( )20mA cm−2,at pH2.7.Plot B:pH (a, )2.7,(b, )2.5,(c,᭹)2.0and(d, )1.8,at10mA cm−2.The electrolyses of300mL of5mM CAA in0.035M Na2SO4with0.5mM FeSO4and H2SO4(to adjust the initial pH)were performed in system II.Anode:Ti/IrO2-Ta2O5.Electrode surface:10cm2.perchlorate,as expected from the production of a higher amount ofoxidizing hydroxyl radicals.In contrast,at20mA cm−2,the chlorideions released during the CAA oxidation were mainly accumulated aschlorate,whereas only a small concentration of perchlorate couldbe detected.Thisfinding is relevant because it suggests that theaccurate selection of the operating parameters such as current den-sity may prevent the accumulation of toxic reaction by-productsupon the electrochemical decontamination process.3.1.4.Oxidation by electrogenerated active chlorineBesides the possible generation of chlorates and perchloratesexposed above,chlorides are expected to be converted intoactiveFig.3.Abatement of CAA using a BDD anode at(a, )5,(b,᭹)10,(c, )20and(d, )40mA cm−2.The electrolyses of300mL of5mM CAA were carried out in0.035M Na2SO4(curves a-d)or0.5M NaCl at40mA cm−2(curve e, )employing system II with continuous recirculation.Cathode:Stainless steel.Electrode surface:10cm2.chlorine(HClO/ClO−and Cl2)at BDD or Dimensionally-Stable Anodes(DSA),which can oxidize the organic pollutants present in the solution[54,55].The abatement of CAA by means of active chlo-rine was assessed by treating solutions of5mM CAA at40mA cm−2 in the presence of0.5M NaCl as supporting electrolyte using the following electrodes:a)Ti/IrO2-Ta2O5electrode as a DSA anode and silver cathodeb)BDD anode and stainless steel cathodeAs mentioned in section3.1.2(Fig.1),in the presence of iridium-based anode and silver cathode,very similar CAA profiles were achieved in the presence of Na2SO4or NaCl as supporting elec-trolyte,thus demonstrating that active chlorine does not contribute in a significant way to the CAA abatement under the adopted oper-ating conditions.On the other hand,the use of NaCl in the BDD/steel system resulted in a drastic decrease of the CAA abatement,as clearly revealed by comparing curves(d-e)in Fig.3.This result was probably due to the waste of a large current percentage in the oxidation of chloride to active chlorine,chlorate and perchlo-rate,which resulted in lower current efficiencies and a slower and poorer CAA abatement because:(i)the oxidation power of these chlorinated species is lower than that of•OH easily formed on BDD in Na2SO4,and(ii)the highly oxidizing•OH are wasted in side reac-tions with chlorinated species to yield chlorinated radicals with a low oxidation power,such as ClOH•−,Cl•and Cl2•−[56].3.1.5.Coupled processSome of the authors have previously shown that the adoption of coupled processes can enhance dramatically the abatement of。
electrochimica acta的endnote -回复

electrochimica acta的endnote -回复关于“electrochimica acta”的问题。
电化学学报(Electrochimica Acta)是一本学术期刊,主要涵盖了电化学及相关领域的研究成果。
该期刊发表了许多重要的研究文章,对电化学和相关学科的发展做出了积极的贡献。
首先,让我们来了解一下电化学的概念。
电化学是研究电与化学之间相互作用的科学,主要探索电子转移、离子输运和电荷传递等过程。
电化学广泛应用于能源存储与转换、材料科学、分析化学和环境科学等领域。
作为电化学领域的顶级学术期刊之一,电化学学报(Electrochimica Acta)收集并发表了大量的研究论文和综述文章,涵盖了电化学和相关学科的各个方面。
这些文章深入研究了电化学反应、电化学技术、电化学分析、电化学界面等方面的问题,并提供了最新的研究进展和应用。
这些文章通过实验、理论和计算模拟等多种方法,深入探索了电化学中的基础科学问题,为电化学领域的进一步发展提供了有益的指导。
从文章的内容来看,电化学学报发表了包括电化学催化、电沉积、电解质、电介质、电分析等方面的文章。
这些文章涵盖了多个学科领域,如能源和电池技术、材料科学和纳米技术、环境和电化学催化等。
具体来说,首先是电化学催化类的研究。
电化学催化是一种通过电流调整化学反应速率的方法。
该研究领域主要关注电化学反应中的催化效应,例如电解水制氢、CO2还原、氧还原反应等。
这些研究成果对于发展可持续的能源转换和存储技术具有重要意义。
其次,电沉积类的研究也是电化学学报中的重要部分。
电沉积是将金属、合金、化合物或纳米结构沉积到电极表面的过程。
这些研究可以提供关于材料生长机理、表面性质和电化学行为的重要信息。
此外,电沉积还广泛应用于材料制备、纳米技术和电化学存储等领域。
另外,电解质、电介质和电分析也是电化学学报中的重要研究方向。
电解质是电解质溶液中的离子传导体,而电介质则是在电极和电解质之间起到绝缘作用的材料。
- 1、下载文档前请自行甄别文档内容的完整性,平台不提供额外的编辑、内容补充、找答案等附加服务。
- 2、"仅部分预览"的文档,不可在线预览部分如存在完整性等问题,可反馈申请退款(可完整预览的文档不适用该条件!)。
- 3、如文档侵犯您的权益,请联系客服反馈,我们会尽快为您处理(人工客服工作时间:9:00-18:30)。
In a typical synthesis and assembly experiment, 30 L of 1% triethylamine toluene solution was carefully dropped and spread onto the surface of 0.7 mL 20 mM Ni(NO3)2 aqueous solution, forming an organic/aqueous interface where an ultrathin film of Ni(OH)2 nanoparticles appeared. The reaction was allowed to last for 1 h. For the preparation of other metal hydroxide, the corresponding metal salt was used.
Huihui Wang a,b, Shilin Zhou a, Zhigang Wang a, Shanling Xu a, Song Dong a, Yuqing Miao a,∗
a School of Science, University of Shanghai for Science and Technology, Shanghai 200093, China b ab of Biomimetic Electrochemistry and Biosensors, Zhejiang Normal University, Jinhua 321004, China
websites are prohibited.
In most cases authors are permitted to post their version of the article (e.g. in Word or Tex form) to their personal website or institutional repository. Authors requiring further information regarding Elsevier’s archiving and manuscript policies are encouraged to visit:
journal homepage: /locate/electacta
Electrochemical conversion of Ni(OH)2 nanoparticle film into nickel hexacyanoferrate through a simple strategy of potential cycling
synthesizing and assembling at gas/liquid interface. The obtained Ni(OH)2 film was transferred onto the glass carbon electrode (GCE) surface and easily derivatized to Ni-HCF during potential cycling in K3[Fe(CN)6] solution.
© 2012 Elsevier Ltd. All rights reserved.
1. Introduction
As an important class of mixed-valence compounds, metal hexacyanoferrates (M-HCFs, including hexacyanoferrate (II) and hexacyanoferrate (III) compounds) have aroused considerable interest due to their attractive physicochemical characteristics of electrochromism, ion exchange, molecular magnetism and electrocatalytic properties [1–4]. As an important material, of low cost, high stability, good catalytic, optical, electronic and magnetic properties, nickel hydroxide Ni(OH)2 has also aroused increasing attention in recent years with its potential applications in electrochromic films, batteries, capacitors, electrochemical sensors etc.[5–7].
abstract
Electrochemical conversion of metal hexacyanoferrates into their oxides or hydroxides was successfully achieved and reported using nickel hexacyanoferrates (Ni-HCF). Herein, a versatile strategy for such a conversion was explored by potential cycling Ni(OH)2 nanoparticle film modified electrodes in K3[Fe(CN)6] or K4[Fe(CN)6] solution. The results show that K3[Fe(CN)6] is more prone to the conversion from Ni(OH)2 to Ni-HCF. Their composition, morphology, and structure were characterized by the techniques such as scanning electron microscopy (SEM), Fourier transform infrared spectroscopy (FTIR) and UV–vis spectrometry. The reaction mechanism is tentatively given. This strategy is also suitable for the other metal hydroxides including rare earth hydroxides such as neodymium, samarium etc.
2. Experimental
2.1. Reagents and materials
Triethylamine, Ni(NO3)2, Nd(NO3)3, Sm(NO3)3, K2[Fe(CN)6] and K3[Fe(CN)6] were from Aladdin (Shanghai, China). All other chemicals were of analytical grade and were used without further purification. All solutions were prepared using ultra-pure water from Milli-Q.
/copyright
Author's personal copy
Electrochimica Acta 74 (2012) 201–206
Contents lists available at SciVerse ScienceDirect
Electrochimica Acta
However to the best of our knowledge, until now it has not been reported about electrochemical conversing metal hydroxides into their HCFs. Herein, a film of Ni(OH)2 nanoparticles was prepared by
article info
Article history: Received 13 October 2011 Received in revised form 16 April 2012 Accepted 16 April 2012 Available online 23 April 2012
Keywords: Ni(OH)2 Hexacyanoferrate Nickel hexacyanoferrate Electrochemical conversion
∗ Corresponding author. Tel.: +86 21 65710112. E-mail addresses: yqmiao@, yqmiao@ (Y. Miao).
0013-4686/$ – see front matter © 2012 Elsevier Ltd. All rights reserved. /10.1016/j.electacta.2012.04.059
This article appeared in a journal published by Elsevier. The attached copy is furnished to the author for internal non-commercial research and education use, including for instruction at the authors institution
Electrochemical conversion of M-HCFs into their oxides or hydroxides has been successfully achieved and reported using nickel hexacyanoferrate (Ni-HCF) [8–10]. A typical strategy is often involved in positively potential cycling Ni-HCF precursor modified electrodes in NaOH or KOH alkali solution where the strong interaction between metal ions and OH− forms M(OH)x, and result in the destruction of the M CN Fe bond. Casella and Gatta [11] also reported the preparation of Ni(OH)2 modified electrodes by electrochemical process in NaOH solution containing K2Ni(CN)4.