g-C3N4共轭纳米结构-光氧化还原
高比表面积gC3N4的合成及光催化研究
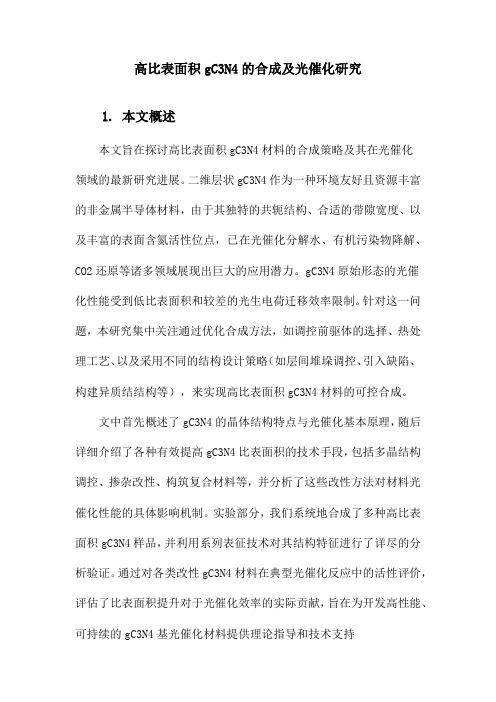
高比表面积gC3N4的合成及光催化研究1. 本文概述本文旨在探讨高比表面积gC3N4材料的合成策略及其在光催化领域的最新研究进展。
二维层状gC3N4作为一种环境友好且资源丰富的非金属半导体材料,由于其独特的共轭结构、合适的带隙宽度、以及丰富的表面含氮活性位点,已在光催化分解水、有机污染物降解、CO2还原等诸多领域展现出巨大的应用潜力。
gC3N4原始形态的光催化性能受到低比表面积和较差的光生电荷迁移效率限制。
针对这一问题,本研究集中关注通过优化合成方法,如调控前驱体的选择、热处理工艺、以及采用不同的结构设计策略(如层间堆垛调控、引入缺陷、构建异质结结构等),来实现高比表面积gC3N4材料的可控合成。
文中首先概述了gC3N4的晶体结构特点与光催化基本原理,随后详细介绍了各种有效提高gC3N4比表面积的技术手段,包括多晶结构调控、掺杂改性、构筑复合材料等,并分析了这些改性方法对材料光催化性能的具体影响机制。
实验部分,我们系统地合成了多种高比表面积gC3N4样品,并利用系列表征技术对其结构特征进行了详尽的分析验证。
通过对各类改性gC3N4材料在典型光催化反应中的活性评价,评估了比表面积提升对于光催化效率的实际贡献,旨在为开发高性能、可持续的gC3N4基光催化材料提供理论指导和技术支持2. 材料制备方法在《高比表面积gC3N4的合成及光催化研究》一文中,“材料制备方法”段落可以这样撰写:本研究采用热缩聚法制备具有高比表面积的二维共轭聚合物gC3N4。
选用三聚氰胺作为前驱体,因其氮含量高且易于热解转化为gC3N4。
具体的制备步骤如下:原料预处理:精确称取适量的高纯度三聚氰胺置于陶瓷舟内,确保无杂质干扰合成过程。
热解过程:将装有三聚氰胺的陶瓷舟放入马弗炉中,在氮气气氛保护下进行程序升温。
初始温度设定为某一低温(如500),随后以一定的升温速率逐渐升至高温(如550600),并在该温度下保温一定时长(比如几小时),促使三聚氰胺发生热解及聚合反应,生成gC3N4。
固相合成g-C3N4及其可见光催化还原水中六价铬

第1期2017年2月No.1 February,2017现代盐化工Modern Salt and Chemical Industry0 引言半导体光催化技术可以在常温常压下利用太阳光激发半导体材料产生光生载流子彻底氧化有机污染物,或将有毒的高氧化数金属离子还原到无毒的低氧化数物质,具有无污染、反应条件温和、可重复利用等优点,是一种治理环境污染和净化水源的理想绿色生产技术。
1972年,Fujishima 和Honda 在n 型半导体TiO 2电极上发现了水的光电催化分解作用[1],标志着环境光催化的起步。
1976年Carey [2]等人的利用TiO 2光催化降解多氯联苯脱氯的实验研究,引领了半导体光催化技术在水体净化研究的进程,以TiO 2为主的环境光催化得以迅速发展。
但禁带宽度Eg~3.2 eV 的TiO 2光谱响应范围较窄(太阳能利用率低)、光量子效率低和易失活的弊端,严重地制约了光催化技术的实际推广应用。
因此,开发新型高效光催化剂和拓宽光催化剂的光响应范围,成为当前光催化领域的研究热点。
C 3N 4是一种共价化合物,具有生物活性、低密度、化学惰性好等优点,备受科研工作者的关注。
Hemley [3]及 Teter 通过计算C 3N 4的带隙和晶体结构等物理性质,提出C 3N 4具有α、β、类立方、立方、石墨相五种不同的结构。
其中石墨相g-C 3N 4材料的硬度最小,常温常压下最稳定,具有电子迁移速率高、耐酸碱腐蚀性强、热稳定性好、氧化能力强以及机械性强等优点。
g-C 3N 4作为一种不含金属的窄带隙半导体(2.7 eV ),能吸收利用太阳能中的可见光,使其广泛用于光催化分解水产氢以及光催化降解污染物等方面。
如Yan [4]等人研究了类石墨相C 3N 4在光催化降解有机物方面的表现,研究结果表明g-C 3N 4的光催化反应活性在某种程度上受到单一半导体量子效率低的影响。
为了解决这个问题,研究者们通过复合、掺杂、改变工艺等提高g-C 3N 4在光催化实验中的活性[5-7]。
g-c3n4还原机理

g-C3N4还原机理一、引言近年来,二维材料的研究引起了广泛的关注。
其中,g-C3N4作为一种重要的二维材料,具有优异的电学、光学和热学性能,在光电器件、传感器、光催化等领域具有广泛的应用前景。
然而,g-C3N4的还原机理尚未被完全揭示,对其还原过程的理解和控制对于优化其性能和应用具有重要意义。
本文将就g-C3N4的还原机理进行探讨。
二、g-C3N4的结构与性质g-C3N4是一种由碳和氮原子构成的二维平面材料,其结构类似于石墨烯。
与石墨烯不同的是,g-C3N4中的碳和氮原子以不同的方式排列,形成了独特的层状结构。
这种结构使得g-C3N4具有良好的热稳定性和化学稳定性,同时具有较高的电子迁移率和可见光吸收能力。
三、g-C3N4的还原过程g-C3N4的还原过程是指通过一定的手段将氧化态的g-C3N4转变为还原态的过程。
这个过程可以通过多种方法实现,如热还原、化学还原、光催化还原等。
在还原过程中,g-C3N4的价带上的电子被激发到导带,同时生成自由电子和空穴。
这些自由电子和空穴在电场的作用下分离,分别迁移到材料的表面。
在迁移过程中,自由电子和空穴会与吸附在g-C3N4表面的物质发生氧化还原反应,从而实现物质的转化和能量的转换。
四、影响因素影响g-C3N4还原过程的主要因素包括温度、气氛、光照条件以及催化剂等。
温度对还原过程的影响主要体现在热力学和动力学两个方面。
气氛对于g-C3N4的还原过程也有重要影响。
在不同的气氛下,参与反应的物质种类和数量会有所不同,从而导致还原过程的差异。
光照条件对g-C3N4的还原过程有重要影响。
在光照条件下,g-C3N4中的电子和空穴被激发,参与到氧化还原反应中。
催化剂对于g-C3N4的还原过程也起着重要作用。
催化剂能够降低反应的活化能,加速反应进程,提高反应效率。
选择合适的催化剂对于优化g-C3N4的性能和应用具有重要意义。
五、实际应用前景由于g-C3N4具有良好的电学、光学和热学性能,其在光电器件、传感器、光催化等领域具有广泛的应用前景。
g-C3N4在能源和环境治理方面的应用

精品文档供您编辑修改使用专业品质权威编制人:______________审核人:______________审批人:______________编制单位:____________编制时间:____________序言下载提示:该文档是本团队精心编制而成,希望大家下载或复制使用后,能够解决实际问题。
文档全文可编辑,以便您下载后可定制修改,请根据实际需要进行调整和使用,谢谢!同时,本团队为大家提供各种类型的经典资料,如办公资料、职场资料、生活资料、学习资料、课堂资料、阅读资料、知识资料、党建资料、教育资料、其他资料等等,想学习、参考、使用不同格式和写法的资料,敬请关注!Download tips: This document is carefully compiled by this editor. I hope that after you download it, it can help you solve practical problems. The document can be customized and modified after downloading, please adjust and use it according to actual needs, thank you!And, this store provides various types of classic materials for everyone, such as office materials, workplace materials, lifestylematerials, learning materials, classroom materials, reading materials, knowledge materials, party building materials, educational materials, other materials, etc. If you want to learn about different data formats and writing methods, please pay attention!g-C3N4在能源和环境治理方面的应用引言:近年来,随着全球能源危机和环境污染问题的不息加剧,寻找可持续进步和环境友好的解决方案成为了一个重要的挑战。
g-C3N4光催化氧化还原性能调控及其环境催化性能增强

g-C3N4光催化氧化还原性能调控及其环境催化性能增强g-C3N4(石墨相氮化碳)是一种新型的二维材料,具有片状结构和较高的光吸收能力,因此在光催化氧化还原性能调控和环境催化性能增强方面具有巨大的潜力。
本文将重点探讨g-C3N4的调控与增强,并分析其在环境催化中的应用。
首先,我们来看g-C3N4的光催化氧化还原性能调控。
光催化氧化还原反应是指在光照下,通过光生载流子的产生和迁移,将底物氧化或还原的反应过程。
g-C3N4作为一种光催化材料,其光催化性能主要受到其能带结构和表面缺陷的影响。
g-C3N4的能带结构中,价带和导带之间的带隙决定了光催化的吸光能力和载流子传输能力。
研究表明,通过控制g-C3N4的合成条件,可以调控其能带结构中的带隙大小和分布,进而调节其光催化性能。
例如,通过控制氮化温度和氮热处理条件,可以提高g-C3N4的带隙大小,使其对可见光的吸收能力增强。
此外,纳米结构和复合材料的调控也可以有效改善g-C3N4的光催化性能。
例如,将g-C3N4与其他半导体纳米材料复合,可以使其能隙气凝胶变窄,光吸收范围增广,从而提高光催化活性。
除了能带结构调控外,表面缺陷也是影响g-C3N4光催化性能的重要因素。
表面缺陷通常是指氮缺陷、碳缺陷和碳氮缺陷等,它们可以促进光生载流子的产生和迁移,提高光催化反应的效率。
因此,通过控制合成条件和引入适量的缺陷,可以增强g-C3N4的光催化活性。
例如,一些研究通过在g-C3N4的合成过程中引入硫、磷等掺杂原子,有效提高了其光催化氧化还原性能。
除了光催化氧化还原性能调控外,g-C3N4还具有良好的环境催化性能,特别适用于污水处理和空气净化等领域。
一方面,g-C3N4作为一种可见光响应的材料,可以通过光氧化、光还原或光催化降解等反应途径,将有机污染物转化为低毒或无毒的无机物。
另一方面,g-C3N4还具有一定的光催化氧化性能,可以将气体污染物如一氧化碳、二氧化氮等转化为无害物质。
g-C3N4光催化氧化还原性能调控及其环境催化性能增强
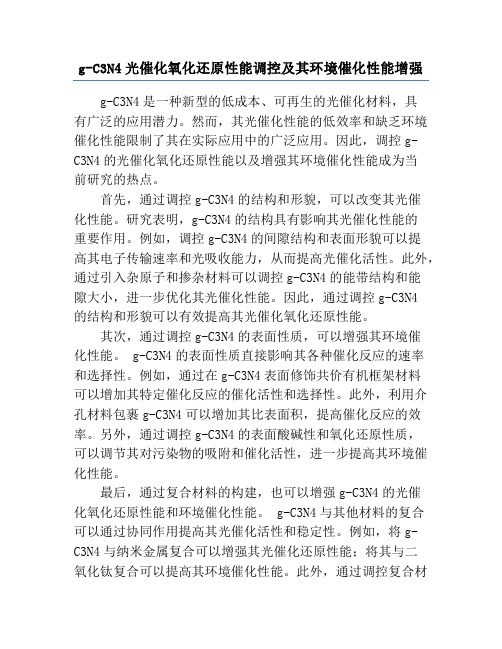
g-C3N4光催化氧化还原性能调控及其环境催化性能增强g-C3N4是一种新型的低成本、可再生的光催化材料,具有广泛的应用潜力。
然而,其光催化性能的低效率和缺乏环境催化性能限制了其在实际应用中的广泛应用。
因此,调控g-C3N4的光催化氧化还原性能以及增强其环境催化性能成为当前研究的热点。
首先,通过调控g-C3N4的结构和形貌,可以改变其光催化性能。
研究表明,g-C3N4的结构具有影响其光催化性能的重要作用。
例如,调控g-C3N4的间隙结构和表面形貌可以提高其电子传输速率和光吸收能力,从而提高光催化活性。
此外,通过引入杂原子和掺杂材料可以调控g-C3N4的能带结构和能隙大小,进一步优化其光催化性能。
因此,通过调控g-C3N4的结构和形貌可以有效提高其光催化氧化还原性能。
其次,通过调控g-C3N4的表面性质,可以增强其环境催化性能。
g-C3N4的表面性质直接影响其各种催化反应的速率和选择性。
例如,通过在g-C3N4表面修饰共价有机框架材料可以增加其特定催化反应的催化活性和选择性。
此外,利用介孔材料包裹g-C3N4可以增加其比表面积,提高催化反应的效率。
另外,通过调控g-C3N4的表面酸碱性和氧化还原性质,可以调节其对污染物的吸附和催化活性,进一步提高其环境催化性能。
最后,通过复合材料的构建,也可以增强g-C3N4的光催化氧化还原性能和环境催化性能。
g-C3N4与其他材料的复合可以通过协同作用提高其光催化活性和稳定性。
例如,将g-C3N4与纳米金属复合可以增强其光催化还原性能;将其与二氧化钛复合可以提高其环境催化性能。
此外,通过调控复合材料的结构和组成,还可以实现对光催化氧化还原性能和环境催化性能的更精确调控。
综上所述,通过调控g-C3N4的光催化氧化还原性能以及增强其环境催化性能可以有效提高其应用潜力。
未来的研究可以进一步探索g-C3N4在其他领域中的应用,并进一步优化其性能,以实现更大的环境益处综上所述,调控g-C3N4的表面性质和构建复合材料是提高其光催化氧化还原性能和环境催化性能的有效方法。
g-c3n4

G-c3n4是一种典型的聚合物半导体,其中CN原子与SP2杂化以形成高度离域的π共轭体系。
g-c3n4的最高占据分子轨道(HOMO)由npz轨道组成,最低未占据分子轨道(LUMO)由CPZ 轨道组成。
带隙约为2.7 EV,可以吸收太阳光谱中波长小于475的蓝紫色光。
·G-c3n4具有非常合适的半导体带边缘位置,可以满足光解氢和氧产生的热力学要求。
·此外,与传统的TiO2光催化剂相比,g-c3n4可有效活化分子氧并产生超氧自由基,可用于有机官能团的光催化转化和有机污染物的光催化降解。
材料历史·C3N4被认为是最古老的合成化合物之一。
它的历史可以追溯到1834年,最早由Berzelius和liebigs报道。
·1922年,富兰克林通过热解Hg(CN)2和Hg(SCN)2等前驱体获得了非晶态氮化碳。
他还提出了其可能的结构。
·1937年,Pauling和Sturdivant首次提出C3N4是一种以共平面三嗪为基本结构单元的多簇化合物,这是通过X射线晶体学证明的。
·在1990年代,C3N4经过长时间的冷却后再次引起了研究人员的注意,但突破主要是在超硬材料β-C3N4的方向上。
·1996年,Teter和hemley用第一原理重新计算了C3N4,并提出C3N4具有五个结构,即α相,β相,C相,P相和G相。
前四个是超硬材料,而g-c3n4是软相,在常温常压下稳定。
·2006年,g-c3n4开始用于非均相催化领域。
福州大学王新臣教授的研究小组在2009年证实,g-c3n4非金属半导体可以在光下催化水产生氢。
·近年来,由于其特殊的结构和优异的性能,g-c3n4成为研究热点,一些企业已成功实现量产,并促进了g-c3n4在光催化领域的商业应用。
超薄g-C3N4纳米片的原位制备及其光催化性能研究
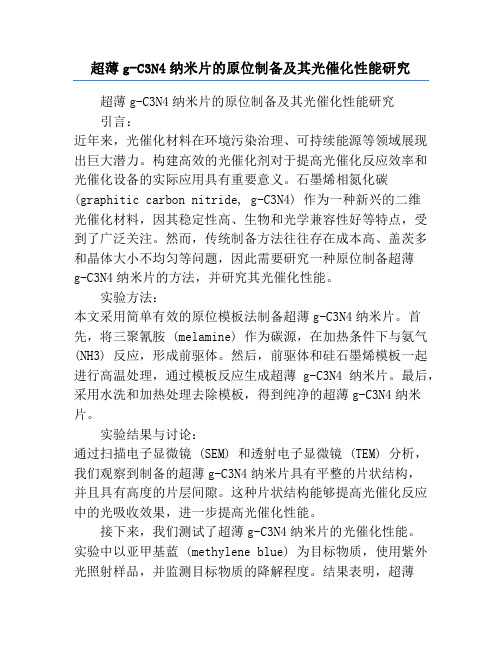
超薄g-C3N4纳米片的原位制备及其光催化性能研究超薄g-C3N4纳米片的原位制备及其光催化性能研究引言:近年来,光催化材料在环境污染治理、可持续能源等领域展现出巨大潜力。
构建高效的光催化剂对于提高光催化反应效率和光催化设备的实际应用具有重要意义。
石墨烯相氮化碳(graphitic carbon nitride, g-C3N4) 作为一种新兴的二维光催化材料,因其稳定性高、生物和光学兼容性好等特点,受到了广泛关注。
然而,传统制备方法往往存在成本高、盖茨多和晶体大小不均匀等问题,因此需要研究一种原位制备超薄g-C3N4纳米片的方法,并研究其光催化性能。
实验方法:本文采用简单有效的原位模板法制备超薄g-C3N4纳米片。
首先,将三聚氰胺 (melamine) 作为碳源,在加热条件下与氨气(NH3) 反应,形成前驱体。
然后,前驱体和硅石墨烯模板一起进行高温处理,通过模板反应生成超薄g-C3N4纳米片。
最后,采用水洗和加热处理去除模板,得到纯净的超薄g-C3N4纳米片。
实验结果与讨论:通过扫描电子显微镜 (SEM) 和透射电子显微镜 (TEM) 分析,我们观察到制备的超薄g-C3N4纳米片具有平整的片状结构,并且具有高度的片层间隙。
这种片状结构能够提高光催化反应中的光吸收效果,进一步提高光催化性能。
接下来,我们测试了超薄g-C3N4纳米片的光催化性能。
实验中以亚甲基蓝 (methylene blue) 为目标物质,使用紫外光照射样品,并监测目标物质的降解程度。
结果表明,超薄g-C3N4纳米片对亚甲基蓝具有良好的光催化降解效果。
此外,我们进一步测试了不同厚度的g-C3N4纳米片的光催化性能,并与传统g-C3N4纳米颗粒进行对比。
实验结果显示,超薄g-C3N4纳米片呈现出更高的光催化活性和降解效率,这可能是由于其较大的比表面积和优良的结构特性所导致的。
结论:本研究成功地采用原位模板法制备了具有高度片层间隙的超薄g-C3N4纳米片,并研究了其光催化性能。
- 1、下载文档前请自行甄别文档内容的完整性,平台不提供额外的编辑、内容补充、找答案等附加服务。
- 2、"仅部分预览"的文档,不可在线预览部分如存在完整性等问题,可反馈申请退款(可完整预览的文档不适用该条件!)。
- 3、如文档侵犯您的权益,请联系客服反馈,我们会尽快为您处理(人工客服工作时间:9:00-18:30)。
Facile in situ synthesis of 2D porous g-C 3N 4and g-C 3N 4/P25(N)heterojunction with enhanced quantum effect for efficient photocatalyticapplicationMingye Ding a ,Wei Wang b ,⇑,Yingjie Zhou b ,Chunhua Lu c ,Yaru Ni c ,Zhongzi Xu caCollege of Materials &Environmental Engineering,Hangzhou Dianzi University,Hangzhou 310018,PR ChinabSchool of Physics and Optoelectronic Engineering,Nanjing University of Information Science &Technology,Nanjing 210044,PR China cCollege of Materials Science and Engineering,Nanjing Tech University,Nanjing 210009,PR Chinaa r t i c l e i n f o Article history:Received 9January 2015Received in revised form 6February 2015Accepted 14February 2015Available online 21February 2015Keywords:Carbon nitride Photocatalysis Quantum effect Heterojunction TiO 2a b s t r a c tThe major challenge of employing photocatalysis for environment protection is to develop high efficient,low cost,and stable semiconductor photocatalysts.In the present study,an in situ annealing strategy is employed for large scale synthesis of two-dimensional (2D)porous graphitic carbon nitride (g-C 3N 4)and efficient g-C 3N 4/P25(N)(N doped P25)heterojunction with enhanced quantum effect.The P25not only serves as the template for g-C 3N 4polymerization,but is also modified by the N species to enhance the visible light pared to the normal bulk g-C 3N 4,the 2D porous g-C 3N 4with enhanced quan-tum effect is found to be more efficient in improving the specific surface area and the electron–hole pair’s separation,even its light absorption edge is blue-shifted.Photocatalytic degradation of Rhodamine B (RhB)and phenol indicates the 2D g-C 3N 4and g-C 3N 4/P25(N)are very efficient and stable under the xenon lamp irradiation.It is also found that the original mass ratio of urea,which is the precursor for g-C 3N 4synthesis and P25modification,to P25also plays a significant effect on the photocatalytic activity.The optimized photocatalyst (mass ratio of P25to urea is 1:8)can decompose total RhB aqueous solution (10mg/L,100ml)in 25min.Based on systematic characterizations and discussions,a possible photocat-alytic mechanism for the excellent photocatalytic performance is proposed.Ó2015Elsevier B.V.All rights reserved.1.IntroductionInorganic semiconductor photocatalysts have been studied widely in the field of environment protection,water splitting,and new fuels synthesis [1–6].Nano-sized photocatalysts often possess unique physical and chemical properties compared to their bulk counterparts.These properties,which are often determined by size,shape,crystallinity,and constitution,often play significant influence on their photocatalytic activity [7].Great research effects have been devoted to preparing nano-sized photocatalysts with defined compositions,morphologies,shapes and fine inner struc-tures.Among these factors,morphologies and shapes have great influence on separating the electrons and holes.For instance,the zero-dimensional (0D)quantum dots (e.g.carbon/CdS/CdSe quan-tum dots)are efficient in enhancing the light absorption and elec-tron–hole pair separation [8,9].One-dimensional (1D)photocatalyst,such as TiO 2and ZnO rods,can promote the fast and efficient electron transportation along the axial direction,hence high performance for solar energy application can be obtained [10,11].Semiconductors with 2D structures are often favorable for heterophotocatalysis because of the shortened per-pendicular distance for photo-generated carriers to migrate from the bulk to the surface.More electrons and holes will participate into the photocatalytic reaction because of the reduced bulk recombination [12–15].In the last several years,g-C 3N 4has been one of the most stud-ied semiconductor photocatalysts because of it is visible light absorption,environment stability,and graphene-like 2D structure,etc.[16–19].A lot of precursors and methods have been applied and various kinds of nano-structured g-C 3N 4have emerged.How-ever,the numerous layers and the relative fast electron–hole pair recombination restrict its photocatalytic activity.Various strate-gies have been applied to overcome this shortcoming [20].For instance,employing thermal treatment and sonication to exfoliate the bulk g-C 3N 4to fewer layers is a very promising idea [21–23].Synthesizing well-structured g-C 3N 4nanospheres by employing porous SiO 2as the template is also reported recently [7].With/10.1016/j.jallcom.2015.02.1110925-8388/Ó2015Elsevier B.V.All rights reserved.⇑Corresponding author.Tel.:+862583587252;fax:+862583587220.E-mail address:wwnjut@ (W.Wang).the help of these methods,the as-prepared few-layered g-C3N4tru-ly exhibits higher photocatalytic performances than the bulk g-C3N4.However,the productivity of these technologies is very low.More efficient methods are needed on this topic.Noble metal deposition,anion atoms(e.g.,O and S)doping,graphene/carbon nanotubes modification are all widely investigated methods to fur-ther improve the photocatalytic activities,but the resulted efficien-cy has not been largely improved[20,24–29].Coupling with semiconductor photocatalyst with a larger band gap to form effi-cient heterojunction is also a promising method,since it can always improve electron–hole separation efficiency and light absorption[30–32].Unfortunately,most of the heterojunction sys-tems are often prepared by physical mixing the two photocatalysts. This strategy often suffers from the drawbacks of uneven mixing and loose contact,thus the performance of the heterojunction can-not be utilized sufficiently.Moreover,practical application of photocatalysts to solve envi-ronment problems requires low cost,large scale production,and environment stable properties.Most of the photocatalysts,such as AgPO4,ZnO,CdS,often suffer serious photocorrosion during the reaction process[33–35].Noble metals and heavy metals are also harmful for the soil and water.To data,TiO2is considered as the most promising candidate for photocatalytic application. Among the industrialized products,Degussa P25is the one with the highest photocatalytic activity because of its unique structure in efficiently separating the electrons and holes.However,the large band gap and the relative high cost restrain its large scale applica-tion.Various methods have been employed to extend the TiO2light absorption edge to the visible region.Among these methods,N doping is the earliest reported and efficient method.However,a green and high efficient method in preparing N doped TiO2is still needed.Furthermore,only this technology cannot make up the above-mentioned shortcomings.In the present study,an in situ and facile annealing strategy is proposed to synthesize2D porous g-C3N4and g-C3N4/P25(N) heterojunction photocatalysts with enhanced quantum effect.Urea is chosen as the precursor both for g-C3N4synthesis and P25 modification.This strategy is a good idea in making sufficient usage of the excellent properties of both g-C3N4and P25.System-atic analysis has evidenced that the2D porous g-C3N4with enhanced quantum effect is much superior to the bulk g-C3N4. Moreover,the heterojunction formed by the2D porous g-C3N4 and P25(N)shows high ability in absorbing the visible light and separating the electrons and holes,resulting in a high and stable photocatalytic activity for phenol and RhB degradation under the xenon lamp irradiation.This study may give a new sight on large scale preparation of efficient g-C3N4-based photocatalysts by inte-grating various processes and factors within one facile but efficient method.2.Experimental2.1.Preparation of bulk g-C3N4Bulk g-C3N4was synthesized with a facial annealing method by employing urea as the precursor.15g of urea was added into a crucible with a cover at room tem-perature,then the crucible was placed into an Muffle furnace and heated at520°C for4h with a temperature ramping rate of25°C/min.The yellow colored sample, named g-C3N4(B),was washed with deionized water to remove the adsorbed impu-rities.At last,g-C3N4(B)was dried and grinded.2.2.Preparation of2D porous g-C3N4and g-C3N4/P25(N)heterojunction photocatalystsStoichiometer amounts of urea(25g)and P25(4.2g)(mass ratio of P25to urea is1:6)were grinded sufficiently in a pot mill with the addition of5ml ethanol.The resulted homogeneous composite contains P25and urea was heated at80°C to eva-porate the ethanol,then the composite was grinded and heated in the Muffle fur-nace similar to that of preparing g-C3N4(B).Thefinal product constituted by P25(N)and2D porous g-C3N4was named g-C3N4(S)–P25(N6)(Scheme1).At last,g-C3N4(S)–P25(N6)was washed with hydrofluoric acid and deionized water to remove the P25(N).The obtained pure2D porous g-C3N4with enhanced quantum effect was named g-C3N4(S).In situ composites with different mass ratios of P25to urea(1:4,1:8,1:10)were also used as the precursors to investigate its effect on the formed heterojunction and the photocatalytic activity.The resulted samples were named g-C3N4(S)–P25(N4),g-C3N4(S)–P25(N8),and g-C3N4(S)–P25(N10).Heterojunction photocatalysts constituted by P25and g-C3N4(B)/g-C3N4(S) were also prepared.In a typical procedure,0.1g of g-C3N4(B)or g-C3N4(S)was mixed with0.3g P25in20ml deionized water under sonication.After centrifuga-tion,the products,named g-C3N4(B)–P25and g-C3N4(S)–P25,were dried and grinded.2.3.CharacterizationPowder X-ray Diffraction(XRD)analysis were taken using an ARL X’TRA diffrac-tometer,a voltage of40kV,at20mA,using a Cu source with K a=0.15406nm.UV–vis diffuse reflectance spectra were measured using a Shimadzu3101spectropho-tometer,using a standard barium sulfate powder as a reference.The reflection mea-surements were then converted to absorption spectra using the Kubelka-Mulk transformation.Fourier Transform Infrared Spectroscopy(FTIR)spectra were col-lected on a Vector-22spectrometer in the wavenumber range of4000–400cmÀ1. Transmission Electron Microscopy(TEM)and Scanning Electron Microscopy (SEM)measurements were conducted using a JEOL JEM-2010and JEOL S-4800, respectively.X-ray Photoelectron Spectra(XPS)were collected on a PHI5000Ver-saprobe system with monochromatic Al K a X-rays.Nitrogen adsorption–desorption isotherms were collected at77K using mMK-tristar3000Surface Area and Porosity Analyzer.Photoluminescence(PL)emission spectra were collected on a FL3-221fluorescence spectrophotometer(excitation wavelength:300nm).2.4.Photocatalytic activity and hydroxide radical(ÅOH)testsPhotocatalytic reactions were carried out in a top-irradiation reaction vessel embraced with the circulating cooling water.In a typical test procedure,50mg of each photocatalyst was added into100ml of the RhB or phenol aqueous solution (10mg/L).Homogeneous suspension was obtained by sonication,and then kept in the dark for1h to establish the adsorption–desorption equilibrium with magnet-ic stirring.At last,the suspension was irradiated by a300W xenon lamp to test the photocatalytic activities.The absorption spectrum of RhB or phenol was analyzed on the UV–vis spectrophotometer every5min after remove the photocatalyst to estimate the degradation efficiency.GeneratedÅOH was analyzed according to our reported procedures,except the RhB or phenol was replaced by terephthalic acid and NaOH[36].3.Results and discussion3.1.Characterization of the photocatalystsFig.1a shows the typical XRD spectra of P25and g-C3N4(S)–P25(N6).All the diffraction peaks are ascribed to P25,and no other diffraction peaks ascribing to g-C3N4or urea are observed.This may because of the complete decomposition of urea and the rela-tive low diffraction intensity of the well-dispersed g-C3N4(S).The intensities of both the diffraction signals are almost the same,indi-cating the aggregation and crystal growth are not serious for P25. According to the Bragg equation,the anatase(001)diffraction peak and rutile(110)diffraction peak of P25shifts to the low angle direction suggests the crystal lattice is expanded,which is the direct evidence of doping P25with N species(Fig.1b)[37].In Fig.1c,the presence of g-C3N4and P25(N)in g-C3N4(S)–P25(N6) is further evidenced by the FTIR analysis.Obvious Ti A O A Ti vibra-tion band shift around500cmÀ1is observed in g-C3N4(S)–P25(N6), indicating impurity atoms(such as N)have been introduced.The breathing vibration at810cmÀ1for triazine unites and the skeletal vibration bands in the range of1200–1600cmÀ1for aromatic CN heterocycles are all the feature bands of g-C3N4,suggesting the successful synthesis of g-C3N4(S)even with the presence of P25 [7,38].With the above analysis,it can be observed that annealing P25with urea is an effective method in synthesizing g-C3N4(S) and modifying P25with N species to extend the light absorption (Fig.1d).As illustrated in Fig.2a,g-C3N4(S)–P25(N6)possesses a con-cave–convex surface morphology,and the g-C3N4(S)is coveredM.Ding et al./Journal of Alloys and Compounds635(2015)34–4035closely on the P25(N)surface.More clear structure is further shown in the TEM image(Fig.2b).It is clear that g-C3N4(S)in g-C3N4(S)–P25(N6)is almost transparent,indicating its few-layered structure.In addition,all the P25(N)is located on g-C3N4(S),which is agreed with Fig.1a.This tight structure is very helpful for improving the heterojunction performance.After removing P25(N)with the hydrofluoric acid,the porous-structured2D g-C3N4(S)was obtained(Fig.2c and d).Large2D plane and clear nanopores with a diameter of ca.30nm,which is agreed with the particle size of P25(Fig.2d inset),in g-C3N4(S)is clearly observed.This structure evidences that the P25(N)particles are truly embedded in g-C3N4(S).It should be clarified that even g-C3N4(S)–P25(N6)does not has the ideal layer upon layer structure,the2D structure of g-C3N4(S)is maintained.Moreover,the hetero-junction is formed all over the P25(N)surface,which is positive for improving the performance.For comparison,g-C3N4(B)was also prepared with the same procedure(Fig.2e).The different mor-phologies of g-C3N4(S)and g-C3N4(B)further evidenced that P25 is an efficient promoter in synthesizing the2D porous g-C3N4(S) with fewer layers.After mixing P25with g-C3N4(B),the resulted g-C3N4(B)–P25has a rough and uneven surface(Fig.2f).Moreover, the P25and g-C3N4(B)are not contacted tightly,which may reduce the heterojunction efficiency.The obtained C/N molar ratio of g-C3N4(S)was ca.0.718from the XPS analysis(Fig.S1),which is close to the theoretic value of fine-structured g-C3N4,suggesting the successful synthesis ofScheme1.Schematic structure of as-prepared2D porous g-C3N4(S)and g-C3N4(S)–P25(N)with enhanced quantum(b)enlarged anatase{101}and rutile{110}diffractions peaks,and(d)UV–vis absorption spectra of P25and g-C3N4(S)–P25(N6); N4(S)–P25(N6).polymeric structure.The C1s XPS spectrum of g-C3N4(S)is present-ed in Fig.S2,the C signals centered at284.6eV and287.9eV are assigned to the sp2C A C bonds of graphitic carbon and the sp2-hy-bridized carbon in N A C@N,respectively,further evidencing the fine g-C3N4framework.Structure differences between g-C3N4(S) and g-C3N4(B)werefirstly investigated by XRD analysis.In Fig.2a,two diffraction peaks belonging to g-C3N4of both the sam-ples centering at13.0°and27.4°are observed,suggesting g-C3N4(S)is stable in the hydrofluoric acid aqueous solution.The much weaker diffraction signal of g-C3N4(S)than that of g-C3N4(S)can be ascribed to the size-dependent properties,since there are fewer layers in g-C3N4(S)and the correlation length of interlayer periodicity of tri-s-triazine building blocks was reduced significantly[7,39].Obviously,the existence of P25can prevent the polymerization process of g-C3N4,hence the few-layered2D por-ous g-C3N4(S)was synthesized.The optical properties of g-C3N4(S)were significantly altered compared to that of g-C3N4(B).In Fig.3b,an obvious blue shift of the absorption edge is observed for g-C3N4(S),and the estimated band gap is increased from2.72eV of g-C3N4(B)to2.85eV of g-C3N4(S)(Fig.3b,inset).Combining with the XRD analysis,we can ascribe these changes to the strong quantum effect,which is com-monly happened when the particle size reduces to several nanometers.For g-C3N4(S),the reduced thickness is the reason for this change,which will give it many excellent properties.More-over,an enhanced light absorption in the visible light region is also observed for g-C3N4(S).The improved mesoporous structure and increased defect sites caused by the layer reduction may be the pri-mary reasons for this variation[40].Accordingly,the specific sur-face area and pore size distribution are also changed.In Fig.3c,a type-IV isotherm with a pronounced H1-type hysteresis loop in the relative pressure of0.4–0.9is clearly observed,convincing there are mesopores located in the polymeric frameworks of g-C3N4(S)[41].The calculated specific surface area and pore size are82m2/g and13nm,respectively,while the corresponding val-ues of g-C3N4(B)is8m2/g and35nm.Fig.3d shows the comparison of N1s XPS spectra.Both g-C3N4(B)and g-C3N4(S)present obvious peaks centering at 398.4eV and400.0eV,which are ascribed to the sp2-hybridized nitrogen in C A N@C and tertiary nitrogen N A(C)3groups[7].Com-bining with the C1s analysis,the existence of both the signals con-vinces the formation of tri-s-triazine which is the key structure of g-C3N4.Moreover,g-C3N4(S)has another obvious peak centered at 401.0eV,corresponding to the N species in C A N A H x.It may result from the enlarged surface area and more exposed C A N A H x groups. However,the N species located in the P25(N)lattice cannot be ana-lyzed because of the relative low content and signal superposition.In the PL emission spectra(Fig.4a),a structure-induced proper-ty change was clearly observed.The PL emission spectra shapes of both g-C3N4(B)and g-C3N4(S)are similar to each other.However, the much lower intensity of g-C3N4(S)proves it can separate the photo-generated electrons and holes more efficiently.This positive effect may be originated from the unique structure of g-C3N4(S), since the few-layered2D sheets can significantly shorten the diffu-sion length for carriers migrate to the surface and promote electron transformation on the plane.Hybrid semiconductorphotocatalysts TEM images of as-prepared photocatalysts.(a,b)g-C3N4(S)–P25(N6);(c,d)g-C3N4(S)and P25(inset);(e)g-C3N4(B);to form heterojunction is helpful for separating photo-generated electrons and holes.The PL intensity of g-C3N4(S)–P25(N6) quenches quickly,indicating that the charge recombination hap-pening in the heterojunction photocatalyst is greatly suppressed, hence,a better photocatalytic performance can be expected.The lowest PL emission intensity of P25is originated from its intrinsic structure(large band gap),which cannot directly prove it has the highest photocatalytic activity.We also investigate theÅOH gen-eration conditions of the as-prepared photocatalysts by employing terephthalic acid as the probe molecule.The typical PL emission spectrum centered at425nm of the resulted solution is shown in Fig.4b.After irradiated the suspensions for the same time,the col-lected PL intensity order is:g-C3N4(S)–P25(N6)>P25> g-C3N4(S)>g-C3N4(B).The result is agreed with that presented in Fig.4a and further evidences the obtained conclusions,since the ÅOH is formed by the reaction by electrons/holes with H2O and A OH,etc.[36].3.2.Photocatalytic tests and mechanismsThe photocatalytic activities of all the photocatalysts were evaluated by RhB(Fig.5a)and phenol(Fig.5b)degradation under the xenon lamp irradiation.Interestingly,g-C3N4(S)is superior to g-C3N4(B)in photocatalysis,and a much enhanced photocatalytic activity is obtained on g-C3N4(S)–P25(N6).The degradation effi-ciency differences between RhB and phenol may because of their different molecule structure,since the organic dyes is often easy to be decomposed.In order to further illustrate the superiorCharacterizations of g-C3N4(B)and g-C3N4(S).(a)XRD patterns;(b)UV–vis absorption spectra and estimated band gaps(inset);(c)N2 corresponding Barrett–Joyner–Halenda pore-size distribution(inset);(d)N1s XPS spectra.emission spectra under the excitation of300nm light and(b)PL emission spectra of2-hydroxyterephthalic acid to analyze the generated N4(S)and g-C3N4(S)–P25(N6).structure of g-C3N4(S),Fig.6shows the RhB degradation efficien-with the presence of g-C3N4(S)–P25and g-C3N4(B)–P25.Obvi-the photocatalytic activity of g-C3N4(S)–P25is higher thang-C3N4(B)–P25.The higher specific surface area and porous structure may give a more efficient charge transfer behavior between g-C3N4(S)and P25.All these results illustrate: 2D porous g-C3N4(S)with fewer layers and enhanced effect is an efficient candidate for photocatalytic application.situ coupling g-C3N4(S)with P25(N)with a facile one-step annealing strategy is very important in forming efficient hetero-junction photocatalyst.Further analysis was applied to investigate theratios of g-C3N4(S)to P25(N)played on the photocatalyticIn the present study,mass ratios of g-C3N4(S)to P25(N)ed by changing the mass ratios of P25to urea.It shouldout that N doping degree of P25will surely be changed,is hard to exactly measure this factor by the XPSP25(N)was employed in all the photocatalyststhe N doped P25.As shown in Fig.7a,the photocatalyticof g-C3N4(S)–P25(N4),g-C3N4(S)–P25(N6),and g-Care increased gradually,while g-C3N4(S)–P25(10)tocatalytic activity than g-C3N4(S)–P25(N8).Hence,weight ratio of urea to P25is8in the present experimentFig.7b shows that the photocatalytic activity of g-Cis very stable after four cycles repetition.This is easybecause P25and g-C3N4are both very stable semiconductors nature environment.Corresponding photocatalytic mechanisms wereshown in Fig.8a.The distance for the excited carriersto the surface of the few-layered2D porous g-C34reduced compared to that of g-C3N4(B),hence more photo-generat-ed carriers can take part in the photocatalytic reactionpose the pollutants.Even g-C3N4(B)can be excitedRhB and(b)phenol degradation efficiency curves with P25,g-C3N4(B),g-C3N4(S),and g-C3N4(S)–P25(N6)under the xenon lampRhB degradation efficiency curves with g-C3N4(B)–P25and g-C3N4(S)–P25the xenon lamp irradiation.degradation efficiency curves with g-C3N4(S)–P25(N4),g-C3N4(S)–P25(N6),g-C3N4(S)–P25(N8),and g-C3N4(S)–P25(N10)under the xenon photocatalytic tests with the presence of g-C3N4(S)–P25(N8).relative valence band and conduction band positions allow the electrons generated on g-C 3N 4(S)migrate to the conduction band of P25(N),and the holes generated on the P25(N)migrate to the valence band of g-C 3N 4(S).Moreover,g-C 3N 4(S)was directly cov-ered on P25(N)surface in the present study,hence a very strong surface interaction is existed and an efficient heterojunction is formed.The N doped impurity level located near the valence band of P25(N)also allows the absorption of visible light for photocat-alytic reaction.As a result,the facile annealing strategy to in situ hybrid g-C 3N 4(S)with P25(N)is helpful to form an effective UV–vis-driven heterojunction photocatalyst.4.Conclusions2D porous g-C 3N 4(S)and g-C 3N 4(S)/P25(N)heterojunction with enhanced quantum effect were in situ prepared by a facile but effi-cient annealing strategy.Structure analysis indicates that P25(N)is an efficient promoter in helping to form the few-layered 2D porous g-C 3N 4(S)which possesses superior properties than the bulk g-C 3N 4(B),even its band gap is enlarged because of the quantum effect.Tight contact between g-C 3N 4(S)and P25(N)is more favor-able for improving the heterojunction performance,and endow them high visible light absorption and electron–hole pair separa-tion efficiency under the xenon lamp irradiation.As a result,g-C 3N 4(S)and g-C 3N 4(S)–P25(N)possess excellent and stable photo-catalytic activities in decomposing phenol and RhB.The properties of g-C 3N 4(S)–P25(N)is optimized by adjusting the mass ratio of P25to urea,and the optimized value is 1:8.Such a facile and in situ annealing strategy may help to provide new insights for the design and preparation of high efficient semiconductor photocatalysts for pollutants degradation.AcknowledgementThis work was supported by the Startup Foundation for Intro-ducing Talent of NUIST (S8113101001).Appendix A.Supplementary materialSupplementary data associated with this article can be found,in the online version,at /10.1016/j.jallcom.2015.02.111.References[1]J.Xing,W.Q.Fang,H.J.Zhao,Chem.–Asian J.7(2012)642–657.[2]A.Kudo,Y.Miseki,Chem.Soc.Rev.38(2009)253–278.[3]Y.Hu,X.Gao,L.Yu,Y.Wang,J.Ning,S.Xu,X.W.D.Lou,Angew.Chem.Int.Ed.52(2013)5636–5639.[4]X.Chen,L.Liu,P.Y.Yu,S.S.Mao,Science 331(2011)746–750.[5]H.Shi,G.Chen,C.Zhang,Z.Zou,ACS Catal.4(2014)3637–3643.[6]J.Lin,Z.Pan,X.Wang,ACS Sustain.Chem.Eng.2(2013)353–358.[7]J.Zhang,M.Zhang,C.Yang,X.Wang,Adv.Mater.26(2014)4121–4126.[8]A.Panneerselvam,M.Green,Nanoscience 1(2012)208–243.[9]Y.F.Wang,A.Hu,J.Mater.Chem.C 2(2014)6921–6939.[10]F.Zuo,K.Bozhilov,R.J.Dillon,L.Wang,P.Smith,X.Zhao,C.Bardeen,P.Feng,Angew.Chem.-Int.Ed.51(2012)6223–6226.[11]X.Wang,G.Liu,G.Q.Lu,H.-M.Cheng,Int.J.Hydrogen Energy 35(2010)8199–8205.[12]W.Tu,Y.Zhou,Z.Zou,Adv.Funct.Mater.23(2013)4996–5008.[13]S.Ida,T.Ishihara,J.Phys.Chem.Lett.5(2014)2533–2542.[14]M.Zhou,X.W.Lou,Y.Xie,Nano Today 8(2013)598–618.[15]Z.H.Liu,Z.Z.Lou,Z.J.Li,G.Wang,Z.Y.Wang,Y.Y.Liu,B.B.Huang,S.Q.Xia,X.Y.Qin,X.Y.Zhang,Y.Dai,mun.50(2014)11046–11048.[16]K.Schwinghammer,M.B.Mesch,V.Duppel,C.Ziegler,J.Senker,B.V.Lotsch,J.Am.Chem.Soc.136(2014)1730–1733.[17]G.Wu,S.S.Thind,J.Wen,K.Yan,A.Chen,Appl.Catal.B 142–143(2013)590–597.[18]J.Chen,S.Shen,P.Guo,P.Wu,L.Guo,J.Mater.Chem.A 2(2014)4605–4612.[19]Q.Zhai,S.Xie,W.Fan,Q.Zhang,Y.Wang,W.Deng,Y.Wang,Angew.Chem.Int.Ed.52(2013)5776–5779.[20]J.Li,B.Shen,Z.Hong,B.Lin,B.Gao,Y.Chen,mun.48(2012)12017–12019.[21]P.Niu,L.Zhang,G.Liu,H.-M.Cheng,Adv.Funct.Mater.22(2012)4763–4770.[22]J.Tian,Q.Liu,A.M.Asiri,A.O.Al-Youbi,X.Sun,Anal.Chem.85(2013)5595–5599.[23]X.Zhang,X.Xie,H.Wang,J.Zhang,B.Pan,Y.Xie,J.Am.Chem.Soc.135(2012)18–21.[24]L.Ge,C.Han,Appl.Catal.B 117–118(2012)268–274.[25]C.Liu,L.Jing,L.He,Y.Luan,C.Li,mun.50(2014)1999–2001.[26]A.Du,S.Sanvito,Z.Li,D.Wang,Y.Jiao,T.Liao,Q.Sun,Y.H.Ng,Z.Zhu,R.Amal,S.C.Smith,J.Am.Chem.Soc.134(2012)4393–4397.[27]S.Obregon,G.Colon,Appl.Catal.B-Environ.144(2014)775–782.[28]B.Chai,T.Peng,J.Mao,K.Li,L.Zan,Phys.Chem.Chem.Phys.14(2012)16745–16752.[29]J.A.Singh,S.H.Overbury,N.J.Dudney,M.Li,G.M.Veith,ACS Catal.2(2012)1138–1146.[30]J.Shi,Chem.Rev.113(2012)2139–2181.[31]L.Zhang,D.Jing,X.She,H.Liu,D.Yang,Y.Lu,J.Li,Z.Zheng,L.Guo,J.Mater.Chem.A 2(2014)2071–2078.[32]S.Chu,Y.Wang,Y.Guo,J.Feng,C.Wang,W.Luo,X.Fan,Z.Zou,ACS Catal.3(2013)912–919.[33]S.Kumar,T.Surendar,A.Baruah,V.Shanker,J.Mater.Chem.A 1(2013)5333–5340.[34]J.Fu,B.Chang,Y.Tian,F.Xi,X.Dong,J.Mater.Chem.A 1(2013)3083–3090.[35]T.Xu,L.Zhang,H.Cheng,Y.Zhu,Appl.Catal.B 101(2011)382–387.[36]W.Wang,C.H.Lu,Y.R.Ni,Z.Z.Xu,CrystEngComm 15(2013)2537–2543.[37]W.Wang,C.H.Lu,Y.R.Ni,Z.Z.Xu,Appl.Catal.B 127(2012)28–35.[38]X.Zhang,H.Wang,H.Wang,Q.Zhang,J.Xie,Y.Tian,J.Wang,Y.Xie,Adv.Mater.26(2014)4438–4443.[39]D.J.Martin,K.P.Qiu,S.A.Shevlin,A.D.Handoko,X.W.Chen,Z.X.Guo,J.W.Tang,Angew.Chem.Int.Ed.53(2014)9240–9245.[40]M.Zhang,J.Xu,R.Zong,Y.Zhu,Appl.Catal.B-Environ.147(2014)229–235.[41]K.S.W.Sing,D.H.Everett,R.A.W.Haul,L.Moscou,R.A.Pierotti,J.Rouquerol,T.Siemieniewska,Pure Appl.Chem.57(1985)603–619.8.Proposed mechanisms of (a)g-C 3N 4(B)and g-C 3N 4(S)in separating the photo-generated electrons and holes;(b)efficient heterojunction of g-C 3N 4(S)–P25(N)absorbing the UV and visible light for photocatalytic application.。