无人机机翼减阻技术研究
基于CST法的新能源无人机翼型优化设计

基于CST法的新能源无人机翼型优化设计余彬; 贺翔; 邓浩【期刊名称】《《航空科学技术》》【年(卷),期】2019(030)010【总页数】6页(P24-29)【关键词】翼型优化; CST方法; 遗传算法; 低雷诺数; 俯仰力矩【作者】余彬; 贺翔; 邓浩【作者单位】北京耐威科技股份有限公司北京 100029【正文语种】中文【中图分类】V211.3新能源无人机是指依靠新能源技术,利用太阳能、氢能等清洁能源,延长续航时间的无污染、高效率的一种无人机。
新能源无人机的运用前景十分可观,军事上能进行长距离持久的军事侦察、巡逻、通信中继等任务;民用上可以进行环境监测、航拍测绘、科研活动等。
新能源无人机已经成为无人机未来的重要发展方向之一。
由于新能源无人机长航时的特点,以及太阳能无人机对于光照的需求,导致其飞行工况多是高空低速的低雷诺数环境。
在高空低速的低雷诺数环境下,翼型的气动效应主要受制于黏性效应的影响,在上下翼面上会发生转捩,出现层流分离泡现象[1],这是低雷诺数翼型的设计计算工作的重点。
在现有的计算流体力学(CFD)数值模拟方法中,直接数值模拟(DNS)和大涡模拟方法(LES)被证实虽然更能模拟现实流动,对小尺度的涡流有着很好的精度,在预估边界层转捩方面具有最好的准确度,但由于这两种方法对于计算机的内存条件的要求过高,通过现有的计算机实现算法过于耗时耗力,很难运用于需要快速高效的工程设计中。
而求解雷诺平均N-S方程方法(RANS方法)相对于DNS和LES对计算机的负担较轻,且具有一定的准确性,可以为大多数层流、湍流工况的流场计算提供足够精确的结果,因此也是最为广泛使用的方法,但对于层流和湍流的混合情况,特别是对于边界层转捩以及分离泡的流动分析,RANS所常用的湍流计算模型均欠缺一定的通用性和准确性。
将LES与RANS方法折中的混合LES/RANS方法理论上能结合二者的优势,但这一方法对于交界处的处理还处在研究阶段,无法应用于工程之中[2]。
跨音速机翼采用鼓包主动减阻技术研究

A方程 模 型 。本 文首 先对鼓 包 控制激 波减 阻 的机 理
进 行 了分 析 , 后 将 该 技 术 应 用 到 了大 型客 机 上 , 然
气 的 排 放 。 因 此 , 一 代 民用 航 空 飞 机 的 设 计 最 下
进 行机 翼跨 音速 减 阻研究
民用 飞 机 设 计 与 研 究
式 中 , P e 和 k代 表密 度 、 强 、 量 、 度 P、、、 压 能 温 和热 传导 系数 , 是 沿 直角 坐标 系 方 向的 速度 分
其中, d为到 物面 的最 近距离 由式 ( ) 示 : 9表
量 性 应 的 量 =( ) , 切 力 分为 / 粘 . t 鲁+ +
在气 动 性 能 方 面 得 到 了相 当 大 的 改 善 , 提 高 效 在
率及 减 少 燃油 消耗 方 面也 取 得 了长 足 的进 步 。从 另 一个 角 度来 说 。 现 有 飞 机 外 形 基 础 上 。 果 没 在 如
对 民航 客机而 言 , 计 巡 航 速 度 大部 分 都 在跨 设 音 速 区域 _ , 2 如何减 少 跨音 速 飞 行 时 的激 波 阻 力 一 ] 直 是飞 机设 计 师 的一 项 重 要 工 作 内容 。本 文采 用 鼓 包 的方 式 对激 波进 行 控 制 以减 少 波 阻 , 高 飞 机 提
= z = “ 多G= “ F , + , 主
: : ,
:
,
多 () 2
0" i 3 1
1
.
1
1
i
 ̄ 3 + a pu ui p 3i
i
i
() e
十c T g 曩
S +
, L 2
() 8
完整版飞行器的阻力和减阻技术
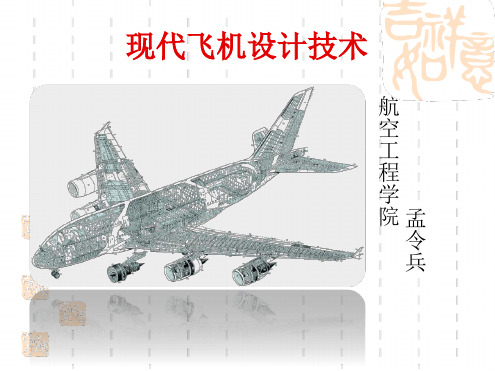
降低干扰阻力的方法:翼身融合
未采用翼身融合技术的米格 21战斗机
降低干扰阻力的方法:翼身融合
未采用翼身融合技术的飞机
(5)激波阻力
? 激波阻力是飞机在空气飞 行过程中产生的一种较强的波 ,由空气遭到强烈的压缩而形 成。当飞行器超声速飞行时, 由于飞行器的能量以强压力波 的形式向周围的空气传递而产 生的一种独特的阻力。激波阻 力对超声速飞行器 翼身组合体 的体积 和横截面积 分布十分敏 感。
现代飞机设计技术
航 空 工 程 学 院孟
令 兵
第四章 飞行器的阻力与减阻技术
第四章 飞行器的阻力与减阻技术
?空气阻力,对于依靠空气升力支撑的飞机 而言,阻力不可避免的负面代价。纵观飞 机的发展史,减阻是永恒的话题。
什么是阻力?
阻力:阻碍运动的力
空气阻力的分类
?空气阻力主要分为: ?压差阻力 ?摩擦阻力 ?诱导阻力 ?干扰阻力 ?激波阻力
降低诱导阻力的方法
3、地效飞行器
降低诱导阻力的方法
3、地效飞行器
(4)干扰阻力
? 机翼、机身、尾翼、发动 机吊舱等,单独放在气流 中所产生的阻力的总和并 不等于整体所产生的阻力 、而是往往小于把它们组 成一个整体时所产生的阻 力。所谓“干扰阻力”就 是飞机各部分之间由于气 流相互干扰而产生的一种 额外阻力。
降低摩擦阻力的方法
?为了减小摩擦阻力,如今比较普遍的做法 是尽可能地提高飞机表面的光滑程度。这 就催生了埋头铆钉等新型零件。此外,摩 擦阻力也与飞机和机翼的尺寸有关。
ห้องสมุดไป่ตู้
提高飞机表面光滑度
(3)诱导阻力
?机翼产生升力的同时,由于 机翼下表面压力大,上表面 压力小,下翼面的高压气流 会绕过两端翼尖 ,力图向翼上 翻,形成翼尖涡。翼尖涡流 使流过机翼的空气产生下洗 速度,此速度有一个向后的 分量,从而产生诱导阻力。
气层减阻技术
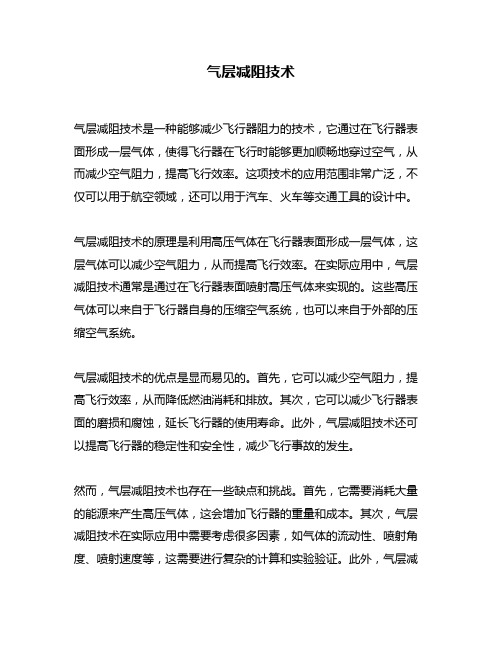
气层减阻技术
气层减阻技术是一种能够减少飞行器阻力的技术,它通过在飞行器表面形成一层气体,使得飞行器在飞行时能够更加顺畅地穿过空气,从而减少空气阻力,提高飞行效率。
这项技术的应用范围非常广泛,不仅可以用于航空领域,还可以用于汽车、火车等交通工具的设计中。
气层减阻技术的原理是利用高压气体在飞行器表面形成一层气体,这层气体可以减少空气阻力,从而提高飞行效率。
在实际应用中,气层减阻技术通常是通过在飞行器表面喷射高压气体来实现的。
这些高压气体可以来自于飞行器自身的压缩空气系统,也可以来自于外部的压缩空气系统。
气层减阻技术的优点是显而易见的。
首先,它可以减少空气阻力,提高飞行效率,从而降低燃油消耗和排放。
其次,它可以减少飞行器表面的磨损和腐蚀,延长飞行器的使用寿命。
此外,气层减阻技术还可以提高飞行器的稳定性和安全性,减少飞行事故的发生。
然而,气层减阻技术也存在一些缺点和挑战。
首先,它需要消耗大量的能源来产生高压气体,这会增加飞行器的重量和成本。
其次,气层减阻技术在实际应用中需要考虑很多因素,如气体的流动性、喷射角度、喷射速度等,这需要进行复杂的计算和实验验证。
此外,气层减
阻技术还需要考虑飞行器的结构和材料,以确保其能够承受高压气体的喷射和飞行时的各种负载。
总的来说,气层减阻技术是一项非常有前途的技术,它可以为航空、汽车、火车等交通工具的设计和制造提供新的思路和方法。
虽然它存在一些挑战和限制,但随着科技的不断进步和发展,相信这项技术将会得到更加广泛的应用和推广。
飞行器的气动设计减阻和增升的基本原理
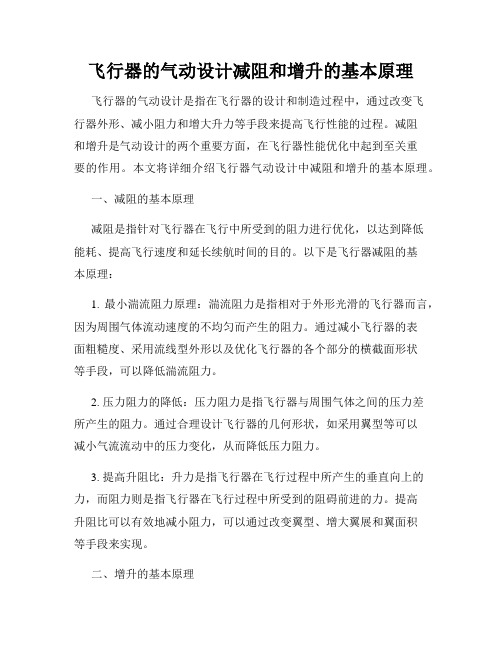
飞行器的气动设计减阻和增升的基本原理飞行器的气动设计是指在飞行器的设计和制造过程中,通过改变飞行器外形、减小阻力和增大升力等手段来提高飞行性能的过程。
减阻和增升是气动设计的两个重要方面,在飞行器性能优化中起到至关重要的作用。
本文将详细介绍飞行器气动设计中减阻和增升的基本原理。
一、减阻的基本原理减阻是指针对飞行器在飞行中所受到的阻力进行优化,以达到降低能耗、提高飞行速度和延长续航时间的目的。
以下是飞行器减阻的基本原理:1. 最小湍流阻力原理:湍流阻力是指相对于外形光滑的飞行器而言,因为周围气体流动速度的不均匀而产生的阻力。
通过减小飞行器的表面粗糙度、采用流线型外形以及优化飞行器的各个部分的横截面形状等手段,可以降低湍流阻力。
2. 压力阻力的降低:压力阻力是指飞行器与周围气体之间的压力差所产生的阻力。
通过合理设计飞行器的几何形状,如采用翼型等可以减小气流流动中的压力变化,从而降低压力阻力。
3. 提高升阻比:升力是指飞行器在飞行过程中所产生的垂直向上的力,而阻力则是指飞行器在飞行过程中所受到的阻碍前进的力。
提高升阻比可以有效地减小阻力,可以通过改变翼型、增大翼展和翼面积等手段来实现。
二、增升的基本原理增升是指在飞行器的设计中,通过改变飞行器的外形和采用一些特殊的设备来增加升力的过程。
以下是飞行器增升的基本原理:1. 翼型的选择:翼型是指飞行器翼的横截面形状,不同的翼型具有不同的升力性能。
选择适合的翼型可以增加翼的升力系数,从而增强飞行器的升力。
2. 翼的迎角:迎角是翼与来流气流的夹角,适当的迎角可以增加翼的升力。
然而,当迎角过大时,会导致翼面分离,产生失速现象。
因此,需要在设计中考虑迎角的合理范围。
3. 辅助升力装置:除了翼型和迎角的选择外,可以通过设计和安装一些辅助升力装置来增加飞行器的升力。
例如,可以安装襟翼、扰流板,或者采用可变角度的前缘襟翼等,来改变气流的流动,增加升力。
结论飞行器的气动设计减阻和增升的基本原理是通过优化飞行器的外形、改善气流流动状态以及采用一些特殊的装置来实现的。
高超声速飞行器减阻防热研究进展
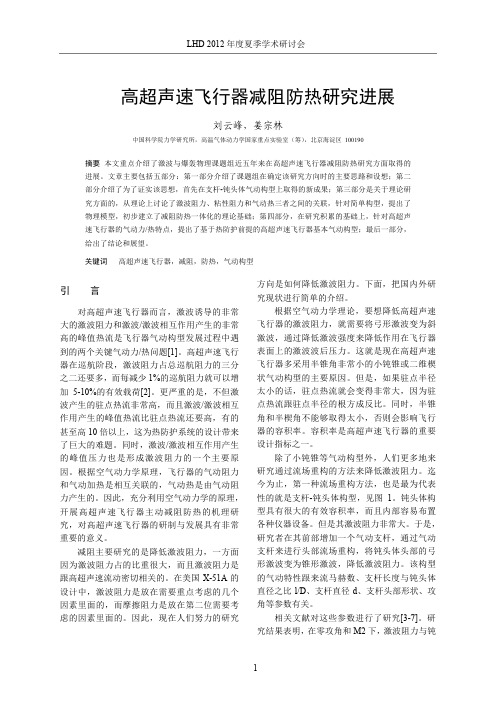
LHD 2012年度夏季学术研讨会高超声速飞行器减阻防热研究进展刘云峰,姜宗林中国科学院力学研究所,高温气体动力学国家重点实验室(筹),北京海淀区 100190摘要本文重点介绍了激波与爆轰物理课题组近五年来在高超声速飞行器减阻防热研究方面取得的进展。
文章主要包括五部分:第一部分介绍了课题组在确定该研究方向时的主要思路和设想;第二部分介绍了为了证实该思想,首先在支杆-钝头体气动构型上取得的新成果;第三部分是关于理论研究方面的,从理论上讨论了激波阻力、粘性阻力和气动热三者之间的关联,针对简单构型,提出了物理模型,初步建立了减阻防热一体化的理论基础;第四部分,在研究积累的基础上,针对高超声速飞行器的气动力/热特点,提出了基于热防护前提的高超声速飞行器基本气动构型;最后一部分,给出了结论和展望。
关键词高超声速飞行器,减阻,防热,气动构型引言对高超声速飞行器而言,激波诱导的非常大的激波阻力和激波/激波相互作用产生的非常高的峰值热流是飞行器气动构型发展过程中遇到的两个关键气动力/热问题[1]。
高超声速飞行器在巡航阶段,激波阻力占总巡航阻力的三分之二还要多,而每减少1%的巡航阻力就可以增加5-10%的有效载荷[2]。
更严重的是,不但激波产生的驻点热流非常高,而且激波/激波相互作用产生的峰值热流比驻点热流还要高,有的甚至高10倍以上,这为热防护系统的设计带来了巨大的难题。
同时,激波/激波相互作用产生的峰值压力也是形成激波阻力的一个主要原因。
根据空气动力学原理,飞行器的气动阻力和气动加热是相互关联的,气动热是由气动阻力产生的。
因此,充分利用空气动力学的原理,开展高超声速飞行器主动减阻防热的机理研究,对高超声速飞行器的研制与发展具有非常重要的意义。
减阻主要研究的是降低激波阻力,一方面因为激波阻力占的比重很大,而且激波阻力是跟高超声速流动密切相关的。
在美国X-51A的设计中,激波阻力是放在需要重点考虑的几个因素里面的,而摩擦阻力是放在第二位需要考虑的因素里面的。
减小激波阻力的措施
减小激波阻力的措施
激波阻力是飞行器在超音速飞行过程中面临的主要挑战之一。
激波阻力的产生源于激波波及飞行器表面时所引起的压力突变,这会导致飞行器受到巨大压力作用,同时增加了空气动力学的阻力。
减小激波阻力是提高飞行器超音速性能的关键之一。
以下是一些减小激波阻力的常见措施:
1. 优化飞行器外形设计:设计合理的外形可以帮助减小激波的产生和阻力的损失。
圆锥体和俯冲设计等外形被广泛用于减小激波阻力,因为这些外形可以更好地分离激波和减小激波对飞行器表面的影响。
2. 使用隐身技术:隐身技术旨在最大限度地减少飞行器的雷达反射面积,从而减小激波阻力的产生。
隐身涂层、减少平面和棱角、采用低反射材料等措施都可以帮助降低飞行器的雷达截面积,从而减小激波阻力。
3. 采用超音速空气动力学理论:超音速空气动力学理论可以帮助优化飞行器的气动外形,以最大程度地减小激波阻力。
通过深入研究和精确计算激波的产生和传播规律,设计合理的减阻措施。
4. 使用航空材料的创新:选择合适的航空材料可以减小激波对飞行器表面的影响。
高强度低密度材料可以在超音速飞行时减小飞行器表面的压力响应,从而减小激波阻力。
5. 采用主动减阻技术:主动减阻技术包括利用电磁激波波导和热电效应等手段控制激波的产生和传播,从而减小激波阻力。
这些技术可以通过调节材料的电磁特性或热特性,降低飞行器受到的激波压力。
综上所述,减小激波阻力是提升飞行器超音速性能的重要任务之一。
通过优化外形设计、使用隐身技术、应用超音速空气动力学理论、采用创新材料和应用主动减阻技术等措施,可以有效降低激波阻力的产生和影响,提高飞行器在超音速飞行时的性能。
无人机技术的研究及应用
无人机技术的研究及应用一、概述无人机技术是一种先进的航空技术,它结合了航空、电子、机械、计算机技术等多个领域,成为未来发展的重要方向。
无人机技术的研究及应用,在国防安全、环境科学、农业农村、交通运输、能源与资源开发等多个领域具有广泛的应用和重大的战略意义。
二、技术性能(一)外形设计无人机的外形设计,直接影响其使用性能和稳定性。
现代飞机的外形设计以实现减阻,减小飞机气动阻力系数为主要目标。
同时,在无人机的实际应用中,其外形设计也需要具备一定的隐蔽性和机动性。
多种材料、结构和制造工艺的差异使得无人机的外形设计具有较大的自由度。
目前,无人机外形设计主流是小型化、轻量化、综合素材化和多任务化。
(二)电子控制电子控制系统是无人机的重要组成部分,它负责控制飞行、航向和姿态等方面。
该系统具有高度的自主性和智能化,可以实现对机体的高效和精准控制。
同时,它还可以实现自主导航和目标探测、跟踪与定位等高级功能。
(三)机动性能无人机的机动性能指的是其在空中运动的能力。
主要包括起降性能、飞行速度、攀升和下降速度、机动灵活性、滞空时间等方面。
无人机的机动性能必须与实际应用场景相匹配,同时不能牺牲其安全性和稳定性。
近年来,无人机的机动性能已经有了非常大的提升,其在常规飞行、特定操作模式、可靠性和稳定性等方面均取得了显著的进步。
三、应用领域(一)军事领域无人机在军事领域的应用主要包括侦察、目标探测、情报收集、打击、情况呈报等。
无人机的高速、高空、长时间、高精度的技术特性适合军事情况下目标锁定和攻击。
该技术对于重要情报侦察、长期实时数据收集和快速反应能力的要求越来越高,多任务无人机的需求逐步增加,使得该领域的无人机应用逐渐扩大。
(二)环境科学领域无人机在环境科学领域的应用主要包括——监测、调查和响应。
空中无人机适用于能源、环境、气候变化等领域的精细化测量和普查,可以获取高分辨率、广域、多变量的数据,有效解决观测地表和大气层的问题。
宽体客机巡航机翼变弯度减阻技术
宽体客机巡航机翼变弯度减阻技术王斌; 郝璇; 郭少杰; 苏诚【期刊名称】《《空气动力学学报》》【年(卷),期】2019(037)006【总页数】9页(P975-983)【关键词】宽体客机; 可变弯度机翼; 减阻; 升阻比; 俯仰力矩; 翼根弯矩【作者】王斌; 郝璇; 郭少杰; 苏诚【作者单位】中国航天空气动力技术研究院北京 100074【正文语种】中文【中图分类】V211.30 引言商用飞机提高燃油效率及降低运营成本,很大程度上取决于发动机性能和外形气动效率。
根据Breguet航程公式,高巡航因子(MaL/D,马赫数与升阻比的乘积)一直是巡航气动设计追求的一个重要目标。
然而,传统民机气动外形即使是采用先进的超临界机翼,仍要承受因兼顾非设计点或其他要求而导致的气动效率损失,主要在于:当前执行的空中交通管制要求飞机在巡航过程中必须采用阶梯巡航方式,这意味着等高定速飞行时,随着燃油减少升力系数逐渐减小,飞行难以保持在恒定的升阻状态;适航条例中对抖振边界的规定要求飞机应能够在大于经济巡航状态的较大速度和升力范围内安全飞行,这使得机翼设计时必须兼顾这些状态下的激波强度控制;巡航外形接近椭圆的展向升力分布有利于降低诱导阻力,而此时较大的翼根弯矩也增大了机翼的结构重量,故总体设计要在气动与重量之间做出权衡;型号系列化发展往往通过延长机身、加强结构和增加发动机推力来实现,这要求机翼在较宽升力范围内均具有足够高的气动潜力,特别是抖振边界特性。
除此之外,气动设计考虑减小配平阻力而对力矩产生限制,或保证干净机翼具有较好的高低速分离特性等因素,都或多或少对巡航升阻比造成影响。
因此,传统巡航外形经过多个设计考虑进行折中后并未达到理想的气动效率,并且只有在特定的飞行剖面下才能获得接近实际最优的性能。
多年来燃油价格的增长以及航空市场对载荷、航程等性能要求的不断提高,持续的系列化发展对飞机设计提出了更高的要求。
国外多个大型飞机制造厂商和研究机构开展了多项变形技术研究,目的就是寻求可经济地提高飞机气动效率和操纵性的潜在技术,其中具有代表性的就是可变弯度机翼技术[1-5]。
第四章 飞行器的阻力和减阻技术
(3)诱导阻力
机翼产生 正升力
上表面P小 下表面P大
空气绕翼尖从下 表面流向上表面
翼尖涡流
(3)诱导阻力
ห้องสมุดไป่ตู้1、翼尖涡流
机翼产生 正升力
上表面P小 下表面P大
空气绕翼尖从下 表面流向上表面
翼尖涡流
迎角越大,机翼上、下表面的压力差越大,翼尖涡流越强。
2、下洗
(3)诱导阻力
V
V
涂层减阻
涂层减阻是在管道或明渠内壁涂上减阻材料以达到 减阻效果的方法, 其关键技术在于减阻涂料和涂敷技 术两个方面。最早应用于石油管道干线输气, 可以使 输运量增加 5%~20%。利用涂层的疏水性, 使得壁面 更光滑, 从而减小了阻力。
高聚合物添加剂减阻
高聚合物添加剂法是近年来减 阻研究的一个重点, 它通过在 流体中溶入少量长链高分子聚 合物来实现减阻。 高聚合物添加剂减阻是通过从 液体内部边界创造条件以实现 减阻, 它们有一个共同的特点 :分子量的量级都高达百万。
降低干扰阻力的方法:翼身融合
未采用翼身融合技术的米格21战斗机
降低干扰阻力的方法:翼身融合
未采用翼身融合技术的飞机
(5)激波阻力
激波阻力是飞机在空气飞 行过程中产生的一种较强的波 ,由空气遭到强烈的压缩而形 成。当飞行器超声速飞行时, 由于飞行器的能量以强压力波 的形式向周围的空气传递而产 生的一种独特的阻力。激波阻 力对超声速飞行器翼身组合体 的体积和横截面积分布十分敏 感。
V
下洗气流速度——下洗气 流垂直向下的分速度。 下洗角——下洗气流与来 流之间的角度。
降低诱导阻力的方法
1、翼梢小翼
降低诱导阻力的方法
1、翼梢小翼
- 1、下载文档前请自行甄别文档内容的完整性,平台不提供额外的编辑、内容补充、找答案等附加服务。
- 2、"仅部分预览"的文档,不可在线预览部分如存在完整性等问题,可反馈申请退款(可完整预览的文档不适用该条件!)。
- 3、如文档侵犯您的权益,请联系客服反馈,我们会尽快为您处理(人工客服工作时间:9:00-18:30)。
American Institute of Aeronautics and Astronautics1Drag Reduction of Light UA V Wing with Deflectable Surfacein Low Reynolds Number FlowsMasoud Darbandi * and Ali Nazari †Sharif University of Technology, Tehran, P.O. Box 11365-8639, Iran Gerry E. Schneider ‡University of Waterloo, Waterloo, Ontario, N2L 3G1, CanadaThe most effective approach to drag reduction is to concentrate on the components that make up the largest percentage of the overall drag. Small improvements on large quantities can become in fact remarkable aerodynamic improvements. Our experience shows that the use of light material in constructing human-powered airplanes and unmanned-air-vehicles UAVs has a few side effects on the aerodynamic characteristics of their wings. One important side effect is the unwanted deflection on wing shell. It is because of high flexibility and low solidity of the light material, which covers the wing skeleton. The created curvature has direct impact on the separation phenomenon occurred over the wing in low Reynolds number flows. In this work, we numerically simulate the flow over a UAV wing with and without considering the generated deflection on its shell. It is shown that the curvature on the wing surface between two supporting airfoil frames causes total drag coefficient reduction. Indeed, this drag reduction is automatically achieved without benefiting from additional drag-reduction devices and/or drag-reduction considerations. The current investigation has been conducted on a UAV wing with fxmp-160 airfoil section. This airfoil normally provides high lift coefficient in low Reynolds flows because of having suitable camber. The drag of a wing with this airfoil section can be reduced by the proper usage of low weight material as its wing shell providing that the wing shell deflects between its supporting frames during stretching the shell in manufacturing stage.Nomenclatureα = angles of attack C d =total drag coefficientC dp =profile drag C ds =skin friction drag C l = two-dimensional lift coefficientC Lthree-dimensional lift coefficient L/D =lift-drag ratio Re = Reynolds numberI. IntroductionRAG reduction is one of the major objectives to the air vehicle designers and manufacturers 1. The study of airvehicles at their cruise shows that there are two main sources of drag force including lift-induced and skin-friction drags. It is reported that these two sources of drag are approximately one-third and one-half of the total drag, respectively, in civil transport aircraft. Reneaux 2 emphasizes that hybrid laminar flow technology and innovates wing tip devices offer the greatest potential for drag reduction. With respect to lift-induced drag, the classical way to reduce drag has been to increase the wing aspect ratio, which is automatically provided in UAV wings. However, for the wings with low aspect-ratio, it is suggested to use various winglet devices such as wing tip sails, wing grid, *Associate Professor, Department of Aerospace Engineering. †Graduate Student, Department of Aerospace Engineering. ‡Professor and Chair, Department of Mechanical Engineering, AIAA Fellow.D3rd AIAA Flow Control Conference5-8 June 2006, San Francisco, CaliforniaAIAA 2006-3680blended winglet, spiroid, and so on2,3. Unfortunately, such unconventional devices cause a complex geometry, which is difficult to be optimized suitably4.In low speed vehicles, laminar flow can be maintained by shaping the airfoil; however, the application of suction in the region of the leading edge has to be applied for high speed vehicle to control the pressure gradient there5. As an alternative strategy, Saric, et al.6 show that artificial roughness can be applied to the leading edge of the wing as a transition control mechanism. Another idea to improve the drag reduction is to use adhesive film coating on wing. Preliminary experiments in the low turbulence wind tunnel at the Langley Research Center has shown that smoothing a wing surface area by applying an adhesive film coating could reduce its drag by as much as 12%, which translated to a 23% reduction in the overall drag of an airplane7. Another mechanism to reduce the drag of fluid is to oscillate the solid wall in the appropriate directions. Jung, et al.8 demonstrated that the skin friction drag of a turbulent channel flow can be reduced by a factor of 40% if one of the walls oscillates in the spanwise direction. Baron and Quadrio9 showed that there would be a 10% net energy saving if the wall was suitably oscillated. Choi, et al.10 and Choi and Clayton11 reached to a similar conclusion with less and more quantifications by investigating on a flat plate. Beside the classical approaches, an emerging technology to control the separation over wing is MEMS, which will effectively contribute to enhance the drag reduction. This technology helps to control the flow through an active manipulation of the coherent structures developed in near-wall region of the boundary layer12.The experience has shown that one primitive idea to reduce the drag of wings is to use flexible wings. The idea has taken into investigation since many decades ago13. Indeed, a flexible aerodynamic surface can be regarded as a possible mean of delaying transition and reducing skin-friction drag. Gyorgyfalvy13 investigated the issue and indicated that the theoretically possible drag reduction is most significant within the Reynolds number ranges from 3 to 50x106 . Hence potential field of flexible wing application would be in sail planes, helicopters, small and medium subsonic airplanes, hydrofoils, torpedoes, speed boats, and miniature submarines. Indeed, the idea of using flexible wings returns to the flow behavior behind the airfoil section utilized in low Reynolds number flow applications. The aerodynamic characteristics of airfoil in low Reynolds number regimes, i.e., Re=5x104 to 1x106, are of importance in a variety of sailplanes and high altitude unmanned aerial vehicles14-15. Tatineni and Zhong14 performed two-dimensional computation over the APEX airfoil and showed that the flow is unsteady with periodic vortex shedding. The vortex shedding is caused by the instability of the separated flow. Redioniottis, et al.16 employed the idea of utilizing an active skin to reduce turbulence drag. The active skin is deformed to take the shape of a traveling wave profile through active material actuation. They indicated that for typical Reynolds numbers encountered during flight condition in UAV’s, the optimum parameters of a surface wave would be a wave length of around 25mm, an amplitude of around 30 µm, and a frequency in the range of a few hundred Hertz. Sinha17 develops a flexible composite surface which is affixed to surfaces of aircraft wings in order to reduce the profile drag. A drag reduction around 18%-37% is reported in flight tests on light aircraft and sailplanes.The wing flexibility issue has been also targeted in wings with low thickness. Shyy, et al.18 treated low Reynolds number environment, i.e., Reynolds as low as 7.5x104 around an elastic, massless membrane as a portion of the upper airfoil surface. They showed that more favorable lift-to-drag characteristics would be obtained when the Reynolds number decreased. Additionally, they deduced that a flexible profile yields better overall performance than a similar rigid profile in an oscillatory free stream. The idea of using curvature of the wing section in improving its aerodynamics characteristics has been similarly touched in the other fields of aerodynamics. For example, Ashil19 utilizes the bump concept and locally (and slightly) modifies the airfoil surface in the shock region. This way, a normal standing shock is weakened into a lambda shock configuration and its strength is reduced by the presence of compression waves.Contrary to the above traditional and innovates drag reduction mechanisms and technologies, we are faced with unwanted deflections on UAV wings20. In reality, the need for designing the light wings for special applications such as UAVs promotes the designer to use light material such as thin plastics to construct its surface shell. The use of such light shells causes unwanted deflections on wing surface. The deflection may be caused by either stretching the skin surface or loading the aerodynamics forces. For example, Deluca, et al.21 investigated a man-portable Micro-Air-Vehicle and showed that the wings would deform considerably under aerodynamics loading. In this work, we focus on the deflection of the wing surface due to surface stretch. The deflection is observed between the two neighboring airfoil sections, located on the wing’s frame, which supports the wing shell. The effect of this deflection is then studied in reducing the wing drag coefficients.2American Institute of Aeronautics and AstronauticsII.Mathematical DescriptionWe choose three-dimensional Navier-Stokes equations to solve the flow field around our UAV wing. The governing equations are discretized using finite-volume method. We choose SIMPLE algorithm to solve the discretized equations. We also use two-equation standard κ-ε turbulence model for turbulence modeling purposes. The near-wall wall-functions are used to model the kinetic energy of turbulence fluctuations κ and its dissipation rate ε near the wall22. The turbulence source terms are approximated at cell faces using power-law scheme. The pressure and diffusion terms are approximated at cell faces using second-order central scheme. However, the convection fluxes at the cell faces are approximated using a second-order upwind scheme23.Since the boundary layer on the airfoil surface and the wake region behind the airfoil play critical roles in the aerodynamic characteristics of the wing, it is necessary to refine mesh in vicinity of wing surface suitably. Additionally, the past investigations have shown that the use of quadrilateral grids in vicinity of the solid walls can tremendously improve the accuracy of the solution. It is because the flow in boundary layer beside the solid wall can be consistently discretized in the flow direction without generating excessive false diffusion in coarse grid employments24. On the other hand, the use of unstructured triangular grid can remarkably reduce the number of cells far form the boundary layer25. To benefit both of these advantages, we have created a hybrid grid topology consisting of a structured quadrilateral grid beside the solid boundary and an unstructured triangular grid for the rest of our computational domain. We have used a multiblocked strategy to distribute these two type of grid topology26,27.Figure 1 partially illustrates the grid distribution around the airfoil section utilized in our UAV wing. Since we intend to verify the accuracy of our numerical solutions against the experimental data, we model the airfoil section in a wind tunnel section. As it is observed, the computational domain is divided by three zones. One structured zone near the wing surface and two unstructured zones in mid and outer regions to the airfoil. Of course, this figure only shows two-dimensional perspective of our three-dimensional grid distribution. Figure 2 shows the deflection occurred on the surface of the wing. Indeed, the deflection on the wing has been exaggerated in order to3American Institute of Aeronautics and Astronauticsprovide a better illustration of what it really happens to its shell. The figure shows the grid between its two consequent airfoils sections (or frames) in the wing. In order to model the curvature, occurred on the wing shell between two supporting frames accurately, we used digitizers. After careful measurements of the deflection in our UAV wing, the deflected surfaces were simulated using the AutoCAD software. In the mesh generation stage, the three-dimensional grids were suitably distributed around the wing considering the created curvature on the wing shell. The grid lines on the wing shell are partially observed in Fig. 2. As it is seen in this close view, the grid on the wing surface is structured and it is clustered wherever it is desired.Fig. 2: A deflection occurred over the wing shell.Since the flow field is solved in an unsteady manner, we consider zero magnitude velocity field and atmospheric pressure in the solution domain as the initial conditions. To be consistent with the conditions implemented in wind tunnel, we numerically modeled the two-dimensional test cases in a channel. We specified the velocity at the inlet and pressure at the outlet. Additionally, we implemented no-slip boundary conditions at the solid walls. We also used wall-function to implement the required boundary conditions for our turbulence governing equations. The preliminary investigation and analysis showed that the walls were far enough not to cause adverse effect on the computed aerodynamic coefficients of the model.III.ResultsAt the first stage of our investigation, it is necessary to verify the computational procedure and to evaluate the accuracy of our algorithm. In this regard, we numerically solve the chosen airfoil section at Re=3x105. It is because the two-dimensional measurements are available at this Reynolds. Figure 3 compares the current numerical solutions with those of measurement for a wide range of angles of attack α. The test have been done for a few angles of attack including 0, 2, 4, 6, 8, 10, 12, and 14. As it is seen, the two lift coefficient distributions agree well within the chosen range of α except for α>12, which is reasonable. This validation ensures us to proceed the rest of our study confidently. The numerical investigation showed that the distribution given in Fig. 3 would be slightly affected in the other close low Reynolds number flows, whose results are not presented here.4American Institute of Aeronautics and Astronautics5American Institute of Aeronautics and AstronauticsIV.ConclusionsThe skin friction and lift-induced are two major sources of drag in air vehicles. The latter drag has minor impact in UAVs because of their large aspect ratio magnitudes of UAV wings. However, skin friction and profile drag play important roles in aerodynamics characteristics of UAV wings because of their flight at low Reynolds. To investigate the impact of wing surface deflection in the aerodynamic performance of the light wing utilized in our6American Institute of Aeronautics and AstronauticsAmerican Institute of Aeronautics and Astronautics7UAV, we arranged a numerical study. Because of the limit in measurements, the code validation was performed at a Reynolds number different from the working one. The investigation showsundesirable because the lift coefficient flight with angles of attack greater Re =generated curvature had also direct impact on drag behavior of our UAV. The current investigation shows that an unwanted deflection on the wing shell of UAV can increase the skin friction. However, the profile drag behavior of the wing in low Reynolds advantages can be utilized to enhance UAVs without the need for removing the occurred deflection. Additionally, this privilege can drastically affect the design of wing’s skeleton and reduce utilized as UAVs’ wing shell.V.REFERENCES1Robert, J.P., “Drag Reduction : An Industrial Challenge,” Special Course on Skin Friction Drag Reduction , AGARD Report 786, 1992. 2Reneaux, J., “Overview on Drag Reduction Technologies for Civil Transport Aircraft,” European Congress on Computational Methods in Applied Sciences and Engineering , ECCOMAS, P. Neittaanmäki, T. Rossi, S. Korotov, E. Oñate, J. Périaux, and D. Knörzer (eds.) Jyväskylä, Finland, 24—28 July 2004. 3Bourdin, P., “Planform Effects on Lift-Induced Drag,” AIAA Paper 2002-3151, The 20th AIAA Applied Aerodynamics Conference, St. Louis, June 24-27, 2002.3Reneaux, J. and Blanchard, A., “The Design and Testing of an Airfoil with Hybrid Laminar Flow Control,” First European Forum on Laminar Flow Technology, Hamburg, Germany, March, 1992.4Grenon, R., and Bourdin, P., “Numerical Study of Unconventional Wing Tip Devices for Lift- Induced Drag Reduction,” CEAS Aerospace Aerodynamics Research Conference Cambridge, June 2002.5Arnal, D., Juillen, J.C., Reneaux, J., and Gasparian, G., “Effect of Wall Suction on Leading Edge Contamination,” Aerospace Science and Technology , No. 8, 1997, pp.505-517.6Saric, W., Carrillo, R., Reibert, M., “Leading-edge Roughness as a Transition Control Mechanism,” AIAA Paper 1998-0781, 36th Aerospace Sciences Meeting and Exhibit , Reno, Nevada, January 1998.American Institute of Aeronautics and Astronautics87Beasley, W.D., and McGhee, R.G., “An Exploratory Investigation of the Effects of a Thin Plastic Film Cover on the Profile Drag of an Aircraft Wing Panel,” NASA TM-74073, October 1977.8Jung, W.J., Mangiavacchi, N., and Akhavan, R., “Suppression of Turbulence in Wall-Bounded Flows by High Frequency Spanwise Oscillations,” Physics of Fluids A , Vol. 4, No. 8, 1992, pp.1605-1607.9Baron, A., and Quadrio, M., “Turbulent Drag Reduction by Spanwise Wall Oscillations,” Applied Scientific Research , Vol. 55, 1996, pp.311-326.10Choi, K.S., DeBisschop, J.R., and Clayton, “Turbulent Boundary Layer Control by Means of Spanwise-Wall Oscillation,” AIAA J., Vol. 36, No.7, 1998, pp.1157-1163.11Choi, K.S., and Clayton, B.R., “ The mechanism of Turbulent Drag Reduction with Wall Oscillation,” International Journal of Heat and Fluid Flow , Vol. 22, 2001, pp.1-9.12Warsop, C., “Current Status and Prospects for Turbulent Flow Control,” AerodynamicDrag Reduction Technologies , Proceedings of the CEAS/DragNet European Drag Reduction Conference, 19-21 June 2000, Springer, Postdam, Germany. 2001.13Gyorgyfalvy, D. “Possibilities of drag reduction in the use of flexible skin,” Journal of Aircraft , Vol.4, No. 3, 1966, pp.186-192.14Tatineni, M., and Zhong, X., “Numerical simulation of unsteady low-Reynolds-number flows over the APEX airfoil,” AIAA paper 1998-0412, 36th Aerospace Science Meeting and Exhibit, Reno, NV, Jan. 12-15, 1998.15Tatineni, M., and Zhong, X., “A numerical study of low-Reynolds-number separation bubbles,” AIAA paper 1999-523, 37th Aerospace Science Meeting and Exhibit, Anaheim, CA, June 11-14, 1999.16Rediniotis, O., Lagouadas, D., Mani, R., Traub, L., and Allen, R. “Computational and experimental studies of active skin for turbulent drag reduction,” AIAA paper 2002-2830, 1st Flow Control Conference, St. Louis, Missouri, 2002.17Sinha, S.K. “Aircraft drag reduction with flexible composite surface boundary layer control, AIAA paper 2004-2121, 2nd AIAA Flow Control Conference, Portland, Oregon, June 28- July 1, 2004.18Shyy, W., Klevebring, F., Nilsson, M., Sloan, J., Carroll, B., and Fuentes, C., “Rigid and flexible low Reynolds number airfoils,” Journal of Aircraft , Vol.36, No.3, 1999, pp.523-529.19Ashil, P.R., Fulker, J.L., and Shires, A., “A Novel Technique for Controlling Shock Strength of Laminar Flow Aerofoil Aections,” First European Forum on Laminar Flow Technology , Hamburg, Germany, March 1992.20Darbandi, M., Nazari, A., and Saeedi, H., “The Aerodynamics of Light Wings with Flexible Surface in Low Reynolds Number Flows,” Proceedings of the Eleventh Asian Congress of Fluid Mechanics May 22-25 2006, Kuala Lumpur, Malaysia. eluca, A.M., Reeder, M.F., Ol, M.V., Freeman, J., Bautista, I., and Simonich, M., “Experimental investigation into the aerodynamic properties of a flexible and rigid wing micro air vehicle,” AIAA paper 2004-2396, 24th AIAA AerodynamicMeasurement Technology and Ground Testing Conference, Portland, Oregon, June 28- July 1, 2004.22Launder, B.E., and Spalding, D.B., “The Numerical Computation of Turbulent Flows,” Computer Methods in Applied Mechanics and Engineering , Vol. 3, 1974, pp.269-289.23Verrsteeg, H.K., and Malalasekera, W. An introduction to Computational Fluid Dynamics; the finite volume method , Addison Wesley Longman Limited, 1995.24Darbandi, M., Schneider, G.E., and Naderi, A.R., “The mesh orientation impact in performance of physical-based upwinding in structured triangular grids,” Proceedings of the 11th Annual Conference of the CFD Society of Canada , CFDSC, Ottawa, ON, Canada, 2003, pp.688-695.25Darbandi, M., Schneider, G.E., and Vakilipour, S., “A Modified Upwind-Biased Strategy to Calculate Flow on Structured-Unstructured Grid Topologies,” AIAA Paper 2004-0435, the 42nd AIAA Aerospace Sciences Meeting and Exhibit, Reno, Nevada, Jan. 5-8, 2004.26Darbandi, M., Schneider, G.E., and Naderi, A.R., “A finite element volume method to simulate flow on mixed element shapes,” AIAA Paper 2003-3638, 36th AIAA Thermophysics Conference, Orlando, FL, June 23-26, 2003.27Darbandi, M., and Naderi, A.R., “Multiblock Hybrid Grid Finite Volume Method to Solve Flow in Irregular Geometries,” Computer Methods in Applied Mechanics and Engineering , in press, 2006.。