水合肼和热还原机理
水合肼的性质及生产工艺

浅谈对水合肼及其工艺技术的认识偶氨二甲酰胺(ADC)是发泡剂的一种,盐湖海虹化工股份有限公司以水合肼和尿素为原料,经缩合、洗涤、氧化等一系列生产工序后制备ADC。
大家对水合肼的了解都较为陌生。
现通过学习对水合肼有了初步认知:1 水合肼的物化性质水合肼(Hydrazine hydrate),又名水合联氨,是肼的一水化物(N2H4·H2O)。
水合肼是无色透明具有发烟的强碱性液体,沸点118.5℃;着火点73 ℃;相对密度1.032;能与水、醇任意混合;不溶于乙醚和氯仿。
有渗透性、腐蚀性,能浸蚀玻璃、橡胶、皮革和软木等。
与氧化剂接触会引起自燃、自爆、有毒、有臭味。
水合肼脱去结合水则形成肼(Hydrazine)N2H4。
肼为油状无色液体,有刺激性的臭味,相对密度1.013,沸点113.5℃,有吸湿性,在空气中发烟。
溶于水、醇、氨、胺;与水能形成共沸物,在碱性溶液中呈现强的还原性。
与卤素、液氨、过氧化氢及其他强氧化剂接触时均可自燃。
长期暴露在空气中或短时期受高温作用,能以爆炸形式分解,贮存时应在氮气中密闭保存。
比水合肼危险性大得多。
水合肼的化学性质来自肼的结构,故肼的化学性质与水合肼的化学性质实质上无差异,其主要化学性质如下:1.1 热分解肼受热分解,产生N2、H2和NH3。
N2H4→N2+2H23N2H4→4NH3+N2N2H4+H2→2NH3金属,如铜、钴、钼及其氧化物,可催化肼的分解过程。
铁锈也能催化分解,在这些催化剂存在下,肼的分解温度明显下降,因此高浓度的肼应贮存于洁净的环境中。
1.2 酸碱性反应肼与水反应呈弱碱性:N2H4+H2O→N2H5+ +OH-N2H4+2H2O→N2H62++2OH-形成正一价肼离子N2H5+和正二价肼离子N2H62+;无水肼与碱金属或碱土金属反应形成肼的金属化物:2Na+2N2H4→2NaN2H3+H2这些肼的离子化物受热或与空气接触,均可引起爆炸。
1.3 还原性反应作为还原剂,肼在碱性溶液中还原能力较亚硫酸强,而弱于亚氯酸;在酸性溶液中的还原能力在Sn3+和Ti2+之间。
水合肼
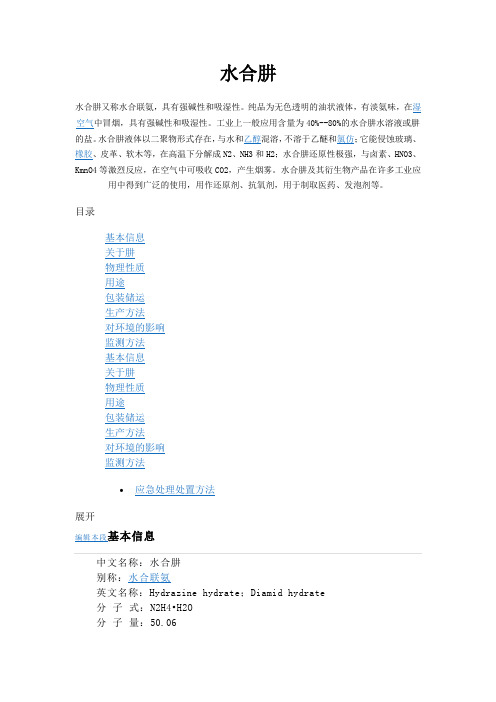
展开编辑本段基本信息中文名称:水合肼别称:水合联氨英文名称:Hydrazine hydrate;Diamid hydrate 分子式:N2H4•H2O分子量:50.06水合肼及其衍生物产品在许多工业应用中得到广泛的使用,如化学产品、医药产品、农化产品、水处理、照相及摄影产品等用作还原剂、抗氧剂,用于制取医药、发泡剂等。
水合肼可直接用作:1.热电厂和核电厂中用作循环水的防腐蚀添加剂。
2.工业锅炉和高压蒸汽炉中用水的除氧剂。
水合联氨是一种脱氧剂,它能使水中的溶解氧还原,被用于进一步去除锅炉给水经热力除氧后的残留微量溶解氧。
因为给水中溶解氧会引起锅炉管壁的腐蚀。
向锅炉给水加入水合肼,不但能脱氧,还能防止锅炉内铁垢和铜垢的生成。
水合肼是一种高效还原剂,可以合成以下产品:1.发泡剂:偶氮甲酰胺(偶氮碳酰胺)(如:用于生产内胎用的叠氮化钠产品)。
2.农化产品和医药产品中生物活性中间体的合成,要先生成三唑。
3.偶氮引发剂4.其它各种产品:在染料方面某些特定的有机颜料产品,照相方面用的试剂,氨基甲酸酯及丙烯酸酯类产品,以及氰溴酸产品。
水合肼还可以在以下领域得到应用:1.贵金属的清洗、精炼。
2.酸洗液和表面处理液中回收金属。
3.处理废液和废气。
4.在电子市场中使用的各等级精制硫酸的提纯。
5.塑料和金属(镍、钴、铁、铬等)的金属镶嵌。
6.是火箭燃料的配方产品。
7.在ACTIRED工艺过程中使用,该工艺主要应用于金矿选矿。
由于水合肼具有双官能基团和亲核基团,因此可以生产多种衍生物产品,如:1. LIOZAN:防腐蚀产品,能提供快速的除氧能力,并且可在较低温度下生效。
2.肼盐:3种产品,主要用于合成中间体(尤其在医药工业中)。
3.三唑产品:1,2,4-三唑/1,2,4-三唑钠盐/4-氨基-1,2,4-三唑/3-氨基-1,2,4-三唑。
4.氨基胍碳酸氢盐。
5.新型的聚合物引发剂,如公司产的液态偶氮化合物产品。
水合肼为强还原剂,是医药、农药、染料、发泡剂、显像剂、抗氧剂的原料;大量用作大型锅炉水的脱氧剂;还用于制造高纯度金属、合成纤维、稀有元素的分离。
邻苯二甲酰亚胺水合肼还原为胺
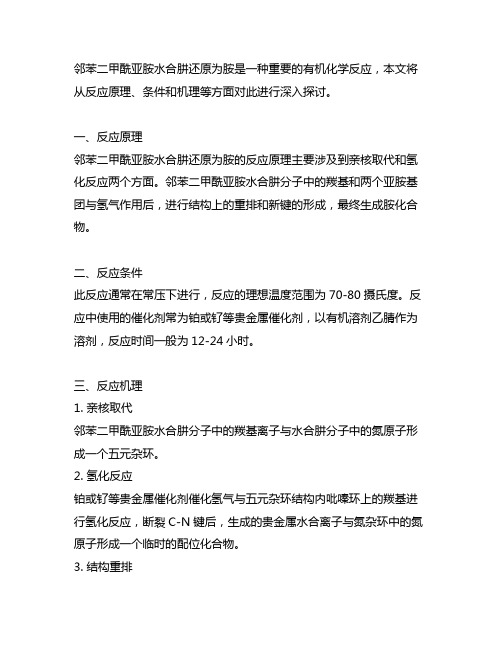
邻苯二甲酰亚胺水合肼还原为胺是一种重要的有机化学反应,本文将从反应原理、条件和机理等方面对此进行深入探讨。
一、反应原理邻苯二甲酰亚胺水合肼还原为胺的反应原理主要涉及到亲核取代和氢化反应两个方面。
邻苯二甲酰亚胺水合肼分子中的羰基和两个亚胺基团与氢气作用后,进行结构上的重排和新键的形成,最终生成胺化合物。
二、反应条件此反应通常在常压下进行,反应的理想温度范围为70-80摄氏度。
反应中使用的催化剂常为铂或钌等贵金属催化剂,以有机溶剂乙腈作为溶剂,反应时间一般为12-24小时。
三、反应机理1. 亲核取代邻苯二甲酰亚胺水合肼分子中的羰基离子与水合肼分子中的氮原子形成一个五元杂环。
2. 氢化反应铂或钌等贵金属催化剂催化氢气与五元杂环结构内吡嗪环上的羰基进行氢化反应,断裂C-N键后,生成的贵金属水合离子与氮杂环中的氮原子形成一个临时的配位化合物。
3. 结构重排贵金属水合离子脱离临时的配位化合物结构,生成新的C=N键。
4. 结构重排新生成的C=N键继续进行水合肼分子中另一个亚胺基团的亲核取代,并与之结合,最终生成胺化合物。
据此反应机理,我们可以通过控制反应条件和催化剂种类等方面,来改进反应的效率和产率。
四、实验方法1. 实验前,根据需要,合成邻苯二甲酰亚胺水合肼;2. 将合成好的邻苯二甲酰亚胺水合肼溶解于适量的有机溶剂中,添加合适的贵金属催化剂;3. 在适当的温度下,通入氢气并搅拌反应物,控制反应时间;4. 反应结束后,通过蒸馏或其他方法提取所需产物。
五、应用和意义邻苯二甲酰亚胺水合肼还原为胺的反应,是合成知名有机合成化合物的重要步骤之一。
已被广泛应用于新药物的合成领域,也对其它有机合成领域有较大的参考意义。
对此反应的深入研究和理解,不仅有助于推动有机合成领域的发展,也具有一定的应用前景。
邻苯二甲酰亚胺水合肼还原为胺的反应原理、条件和机理等方面已有较深入的探讨,这为进一步优化该反应提供了重要的理论依据。
该反应在有机合成领域的应用前景值得进一步的探索和开发。
水合肼分子式

水合肼分子式水合肼,又称肼水合物,是一种化学物质,化学式为N2H4·xH2O,其中x表示水合物的个数。
肼是一种无色液体,在常温常压下为挥发性液体,具有刺激性气味。
肼具有较强还原性,易燃易爆。
水合肼的分子式中的x可以是0、1或2。
当水合肼无水时,化学式为N2H4。
水合肼的结构中存在着一个或两个水分子。
这两种不同的水合肼分子式都有着相应的物理和化学性质。
将水合肼加热至100℃以上,水合肼会分解为氨气和一氧化二氮,这是一种剧烈的分解反应,会伴随剧烈的爆炸。
因此,在储存和处理水合肼时必须小心谨慎,避免产生意外和危险。
水合肼具有较强的还原性,在化学反应中常常用作还原剂。
它可以还原一些金属离子,如银离子、铁离子等。
水合肼的还原性也使其成为合成其他化合物的重要中间体。
例如,通过将水合肼与硝酸银反应,可以制备出肼合银,用于制备炸药。
水合肼还常用作航空航天和火箭发动机燃料的一部分。
在这些应用中,水合肼的能量密度高,易于储存和加工,因此被广泛应用于推进剂的制备中。
然而,水合肼也具有较高的毒性,对人体和环境有一定的危害,因此在使用时需要注意安全问题。
在水合肼的降解和代谢过程中,会产生一些有害物质,如亚硝酸盐。
因此,在使用水合肼推进剂时,需要考虑相应的环境和健康风险,并采取相应的防护措施。
除了以上的应用和风险,水合肼还可用于电镀、催化剂和药物合成等方面。
在电镀中,水合肼可用作一种清洁剂,用于净化金属表面。
在催化剂的制备中,水合肼可用作还原剂或保护剂。
在药物合成中,水合肼可以作为羧酸保护基的一种试剂,用于保护羧基。
水合肼分子式的研究对我们理解其化学性质和应用具有重要意义。
研究水合肼的分解反应和降解机制,可以帮助我们更好地了解其使用中的风险和安全问题。
此外,研究水合肼的合成方法和改性技术,可以进一步扩展其应用领域和提高其性能。
总之,水合肼是一种重要的化学物质,具有较强的还原性和能量密度。
尽管其在航天、火箭发动机等领域有着广泛的应用,但在使用时需要注意安全问题,并采取相应的预防和保护措施。
水合肼反应后处理-概述说明以及解释
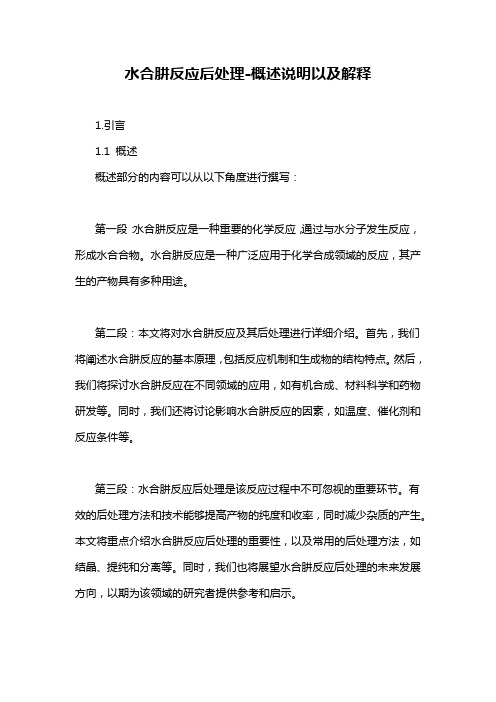
水合肼反应后处理-概述说明以及解释1.引言1.1 概述概述部分的内容可以从以下角度进行撰写:第一段:水合肼反应是一种重要的化学反应,通过与水分子发生反应,形成水合合物。
水合肼反应是一种广泛应用于化学合成领域的反应,其产生的产物具有多种用途。
第二段:本文将对水合肼反应及其后处理进行详细介绍。
首先,我们将阐述水合肼反应的基本原理,包括反应机制和生成物的结构特点。
然后,我们将探讨水合肼反应在不同领域的应用,如有机合成、材料科学和药物研发等。
同时,我们还将讨论影响水合肼反应的因素,如温度、催化剂和反应条件等。
第三段:水合肼反应后处理是该反应过程中不可忽视的重要环节。
有效的后处理方法和技术能够提高产物的纯度和收率,同时减少杂质的产生。
本文将重点介绍水合肼反应后处理的重要性,以及常用的后处理方法,如结晶、提纯和分离等。
同时,我们也将展望水合肼反应后处理的未来发展方向,以期为该领域的研究者提供参考和启示。
总结起来,本文将全面论述水合肼反应及其后处理的相关内容,旨在加深对该反应的理解和应用,为相关领域的科研工作者提供有益的知识和技术支持。
通过深入研究水合肼反应及其后处理,我们可以不断提升该反应的效率和产物的质量,推动相关领域的发展与创新。
1.2文章结构文章结构:本文将按照以下结构进行阐述水合肼反应后处理的内容:1. 引言1.1 概述:介绍水合肼反应及其在化学领域的重要性和广泛应用。
1.2 文章结构:详细说明文章的整体结构,包括正文和结论部分的内容安排。
1.3 目的:阐明本文旨在探讨水合肼反应后处理的重要性及其可能的未来发展方向。
2. 正文2.1 水合肼反应的基本原理:解释水合肼反应的基础知识,包括反应机理和关键步骤。
2.2 水合肼反应的应用领域:探讨水合肼反应在化学和相关领域的广泛应用,并举例说明其在合成和催化等方面的具体应用。
2.3 水合肼反应的影响因素:分析影响水合肼反应后处理效果的因素,包括反应条件、催化剂选择等,以及这些因素对反应结果的影响。
芳香族硝基化合物的水合肼催化还原反应的研....
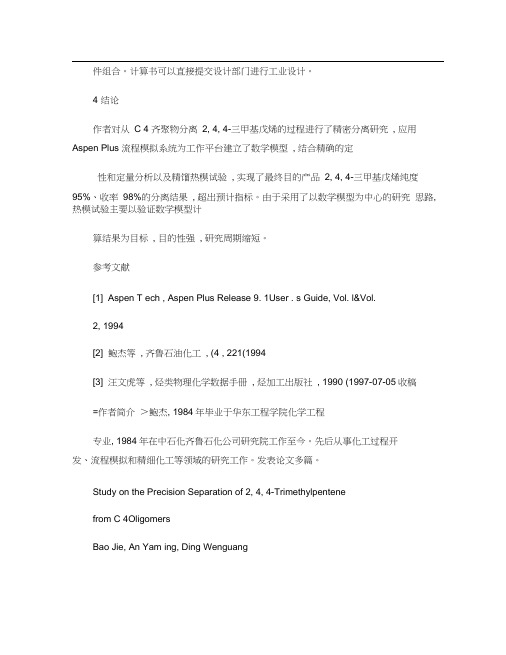
件组合。
计算书可以直接提交设计部门进行工业设计。
4 结论作者对从 C 4 齐聚物分离2, 4, 4-三甲基戊烯的过程进行了精密分离研究, 应用Aspen Plus 流程模拟系统为工作平台建立了数学模型, 结合精确的定性和定量分析以及精馏热模试验, 实现了最终目的产品2, 4, 4-三甲基戊烯纯度95%、收率98%的分离结果, 超出预计指标。
由于采用了以数学模型为中心的研究思路, 热模试验主要以验证数学模型计算结果为目标, 目的性强, 研究周期缩短。
参考文献[1] Aspen T ech , Aspen Plus Release 9. 1User . s Guide, Vol. l&Vol.2, 1994[2] 鲍杰等, 齐鲁石油化工, (4 , 221(1994[3] 汪文虎等, 烃类物理化学数据手册, 烃加工出版社, 1990 (1997-07-05收稿=作者简介>鲍杰, 1984年毕业于华东工程学院化学工程专业, 1984年在中石化齐鲁石化公司研究院工作至今。
先后从事化工过程开发、流程模拟和精细化工等领域的研究工作。
发表论文多篇。
Study on the Precision Separation of 2, 4, 4-Trimethylpentenefrom C 4OligomersBao Jie, An Yam ing, Ding Wenguang(Qilu Petr ochemical Research I nstitute, Zibo , Shandong Pr ovince, Pos tcode 255400Abstract:The precision separation of 2, 4, 4-trimethy lpentene from C 4oligomers w as studied. The flowsheet simulation model of the process in Aspen Plus platform w as built up and the accurate analysis and a few bench -tests w ere accomplished. The results show ed that the purity of 2, 4, 4-tirmethy lpentene w as over 95%and the recovery yield reached 98%.Keywords:oligomers, 2, 4, 4-trimethylpentene, precision separation, Aspen Plus芳香族硝基化合物的水合肼催化还原反应的研究唐洪张蕾徐寿颐(清华大学化学系, 北京, 邮编100084摘要研究了以80%水合肼为还原剂,在FeCI 3#6H 20/C存在下,回流3~8h,将芳香族硝基化合物转变成芳香族氨基化合物的还原反应。
水合肼湿法还原金试验研究
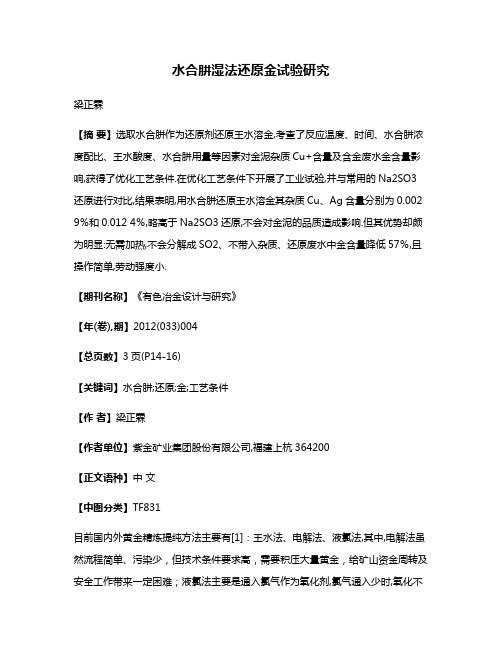
水合肼湿法还原金试验研究梁正霖【摘要】选取水合肼作为还原剂还原王水溶金,考查了反应温度、时间、水合肼浓度配比、王水酸度、水合肼用量等因素对金泥杂质Cu+含量及含金废水金含量影响,获得了优化工艺条件.在优化工艺条件下开展了工业试验,并与常用的Na2SO3还原进行对比,结果表明,用水合肼还原王水溶金其杂质Cu、Ag含量分别为0.002 9%和0.012 4%,略高于Na2SO3还原,不会对金泥的品质造成影响.但其优势却颇为明显:无需加热,不会分解成SO2、不带入杂质、还原废水中金含量降低57%,且操作简单,劳动强度小.【期刊名称】《有色冶金设计与研究》【年(卷),期】2012(033)004【总页数】3页(P14-16)【关键词】水合肼;还原;金;工艺条件【作者】梁正霖【作者单位】紫金矿业集团股份有限公司,福建上杭364200【正文语种】中文【中图分类】TF831目前国内外黄金精炼提纯方法主要有[1]:王水法、电解法、液氯法,其中,电解法虽然流程简单、污染少,但技术条件要求高,需要积压大量黄金,给矿山资金周转及安全工作带来一定困难;液氯法主要是通入氯气作为氧化剂,氯气通入少时,氧化不彻底,影响回收率,氯气通人多时,逸出的氯气对人体有害。
王水法具有操作简单、回收率高等优点得到广泛应用。
其工艺均采用王水溶金,再加入还原剂将金还原,或加入掩蔽剂络合干扰物质,再进行萃取提取。
因此,还原剂的选择尤为重要,直接决定了黄金提纯的质量和成本。
用于金氯酸溶液还原的还原剂种类很多[2,3]:有草酸、抗坏血酸、甲醛、氢醌、水合肼、二氧化硫、硫酸亚铁、亚硫酸钠等。
生产实践中根据金溶液中的含杂质情况选择还原剂种类,必要时选择还原能力强弱不同的两种或多种还原剂组合还原,以产生较佳还原效果。
目前工业主要使用亚硫酸钠作为还原剂进行还原。
但用亚硫酸钠作还原剂时需加热至75℃左右,耗费能源,在还原过程中还会产生大量SO2污染环境,且还原时相应的会引入少量的杂质,如钠离子在还原过程中生成相应的Na2SO4和NaCl等盐类,并进入金粉中影响金泥质量。
水热还原法制银纳米颗粒
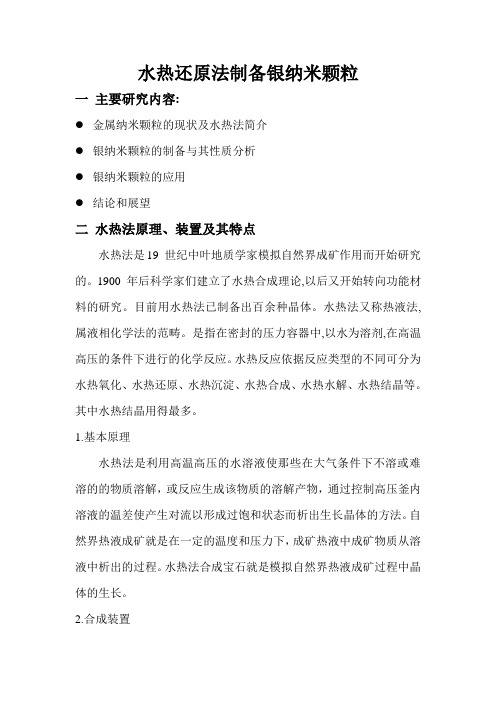
水热还原法制备银纳米颗粒一主要研究内容:●金属纳米颗粒的现状及水热法简介●银纳米颗粒的制备与其性质分析●银纳米颗粒的应用●结论和展望二水热法原理、装置及其特点水热法是19 世纪中叶地质学家模拟自然界成矿作用而开始研究的。
1900 年后科学家们建立了水热合成理论,以后又开始转向功能材料的研究。
目前用水热法已制备出百余种晶体。
水热法又称热液法,属液相化学法的范畴。
是指在密封的压力容器中,以水为溶剂,在高温高压的条件下进行的化学反应。
水热反应依据反应类型的不同可分为水热氧化、水热还原、水热沉淀、水热合成、水热水解、水热结晶等。
其中水热结晶用得最多。
1.基本原理水热法是利用高温高压的水溶液使那些在大气条件下不溶或难溶的的物质溶解,或反应生成该物质的溶解产物,通过控制高压釜内溶液的温差使产生对流以形成过饱和状态而析出生长晶体的方法。
自然界热液成矿就是在一定的温度和压力下,成矿热液中成矿物质从溶液中析出的过程。
水热法合成宝石就是模拟自然界热液成矿过程中晶体的生长。
2.合成装置高压釜为可承高温高压的钢制釜体。
水热法采用的高压釜一般可承受11000C的温度和109Pa的压力,具有可靠的密封系统和防爆装置。
高压釜的直径与高度比有一定的要求,对内径为100-120mm的高压釜来说,内径与高度比以1:16为宜。
高度太小或太大都不便控制温度的分布。
由于内部要装酸、碱性的强腐蚀性溶液,当温度和压力较高时,在高压釜内要装有耐腐蚀的内衬(贵金属如铂金或黄金内衬或是一些高分子聚合物),,以防矿化剂与釜体材料发生反应。
也可利用在晶体生长过程中釜壁上自然形成的保护层来防止进一步的腐蚀和污染。
如合成水晶时,由于溶液中的SiO2与Na2O和釜体中的铁能反应生成一种在该体系内稳定的化合物,即硅酸铁钠(锥辉石NaFeSi2O6 acmite)附着于容器内壁,从而起到保护层的作用。
3、水热法的特点:a)合成的晶体具有晶面,热应力较小,内部缺陷少。
- 1、下载文档前请自行甄别文档内容的完整性,平台不提供额外的编辑、内容补充、找答案等附加服务。
- 2、"仅部分预览"的文档,不可在线预览部分如存在完整性等问题,可反馈申请退款(可完整预览的文档不适用该条件!)。
- 3、如文档侵犯您的权益,请联系客服反馈,我们会尽快为您处理(人工客服工作时间:9:00-18:30)。
Hydrazine and Thermal Reduction of Graphene Oxide:Reaction Mechanisms,Product Structures,and Reaction DesignXingfa Gao,†Joonkyung Jang,‡and Shigeru Nagase*,†Department of Theoretical and Computational Molecular Science,Institute for Molecular Science,Myodaiji,Okazaki 444-8585,Japan,and Department of Nanomaterials Engineering,Pusan National Uni V ersity,Miryang,627-706,Republic of Korea Recei V ed:September 27,2009The density functional theory method (M05-2X/6-31G(d))was used to investigate reaction mechanisms for deoxygenation of graphene oxides (GOs)with hydrazine or heat treatment.Three mechanisms were identified as reducing epoxide groups of GO with hydrazine as a reducing agent.No reaction path was found for the hydrazine-mediated reductions of the hydroxyl,carbonyl,and carboxyl groups of GO.We instead discovered the mechanisms for dehydroxylation,decarbonylation,and decarboxylation using heat treatment.The hydrazine de-epoxidation and thermal dehydroxylation of GO have opposite dependencies on the reaction temperature.In both reduction types,the oxygen functionalities attached to the interior of an aromatic domain in GO are removed more easily,both kinetically and thermodynamically,than those attached at the edges of an aromatic domain.The hydrazine-mediated reductions of epoxide groups at the edges are suspended by forming hydrazino alcohols.We provide atomic-level elucidation for the deoxygenation of GO,characterize the product structures,and suggest how to optimize the reaction conditions further.1.IntroductionWhen sp 2-carbon atoms are arranged into two-dimensional fused hexagons,they form a single-atom-thick allotrope of carbon:graphene.Graphene has been known as the building block of graphite for more than 60years,1but its experimental isolation was first achieved in 2004.2Despite its short history of realization,graphene has attracted much attention because ofitsintriguingcrystalline,mechanical,andelectronicproperties.3–5The measurements of elastic properties and intrinsic breaking strength have established graphene as the strongest material ever measured.6Reportedly,it has an ultrahigh surface area to mass ratio,7and an intrinsic charge mobility that is higher than any semiconductor reported to date.8Its band gap 9,10and magnetic properties 11,12can be tailored by changing its edge structure and by chemical modification of its interior or edges.These features have made graphene and graphene derivatives ideal for diverse applications such as energy-storage capacitors,13sensors,14and transistors.15–18Graphene has emerged as a “shining star”in materials science.3–5It remains as a challenge to produce high-quality graphene on a large scale.Several laboratory methods have been proposed,each with its own advantages and shortcomings.2,18–23Among them,the chemical reduction of graphene oxide (GO)yielding chemically modified graphene (CMG)has intriguing features:it is cheap and easily scalable to a high-volume production.Particularly,being a solution-based approach,it is well-suited for chemical modification and subsequent processing.18,19Con-sequently,the reduction of GO is widely used for device applications such as preparation of graphene-based composites,thin films,and paper-like materials.13,14,18,22,24–36Optimization of reaction conditions has revealed that hydrazine treatment and thermal annealing of GO provide good efficiency for deoxy-genation (Table 1).17,25,36a,37–40It has also been reported that reduction of GO with NaBH 4followed by treatment with concentrated sulfuric acid and thermal annealing at 1100°C yields CMG with high purity.36bOxygen functionalities of four kinds are known to exist in GO:epoxide (-O -),hydroxyl (-OH),carbonyl (-C d O),and carboxyl (-COOH).41–43Epoxide and hydroxyl,located on the basal plane of GO,are the major components;carbonyl and carboxyl,distributed at the edges of GO,are minor.41–43Gao et al.recently suggested that the presence of five-membered-ring and six-membered-ring lactols at the edges of GO are most likely responsible for the minor oxygen-containing impurities of GO.36b After a separate treatment with hydrazine and heat,the oxygen atoms in GO are greatly reduced (Table 1).The CMG obtained from the chemical reduction differs markedly from a defect-free graphene.2The CMG includes,apart from the structural disorder and defects inherited from the pristine GO,a large amount of residual oxygen functionality.35,44–49For hydrazine reduction,the CMG also contains a considerable amount of N-containing species (Table 1).47a,48,49Experimental characterization of the CMG structure and the reduction mechanisms of GO are severely hampered by the amorphous nature of CMG.Stankovich et al.47a proposed that the hydrazine-assisted de-epoxidation of GO follows the mechanism shown in Scheme 1.This mechanism nevertheless requires experimental or computational verification.Recently,Kim et al.have studied the epoxide reduction with hydrazine on graphene.47b It is also necessary to clarify the reduction mechanisms for other oxygen functionalities of GO.It remains unclear whether the hydroxyl group is removed in the reduction of GO with hydrazine.47a The reaction temperature greatly influences the deoxygenation efficiency of GO,49but the underlying mechanism is not known.To improve production of graphene-based functional materials via chemical reduction,it is crucial to understand the structure(s)of CMG sheets and their reaction mechanisms.19*To whom correspondence should be addressed.E-mail:nagase@ims.ac.jp.†Institute for Molecular Science.‡Pusan National University.J.Phys.Chem.C 2010,114,832–84283210.1021/jp909284g 2010American Chemical SocietyPublished on Web 12/04/2009In this article,using the density functional theory method,we investigate the reactions of four oxygen functionalities of GO systematically under hydrazine and heat treatment condi-tions.More specifically,attempts are made to answer the questions presented in Figure 1.19Our results provide atomistic details for deoxygenation of GO via hydrazine and heat treatments.We also suggest methods to optimize the deoxy-genation conditions of GO.putational Details2.1.GO Models.Experimentally available GOs are amor-phous materials with large sizes.Their stoichiometries and structures vary depending on the methods used for their syntheses.41,47a,50Microscopic image 44,45,51and Raman spectros-copy 28have shown that oxygen functionalities form islands and lines on the basal plane of GO,dividing the GO sheet into small in-plane aromatic domains (Figure 2).Therefore,we model the local surface structures of GO as fragmental graphene sheets modified with oxygen functionalities.A similar model has been used to compute the erosion and combustion of graphite.The model was verified as reliable through comparison with experiments.51,52We investigated the surface-size effects on the reduction of GO by systematically increasing the model sheet size.The epoxide groups of GO are classified into two types:epoxides that are located at the interior of an aromatic domain of GO,and epoxides located at the edges of an aromatic domain.The edges are an important determinant of the reactivity of graphene nanosheets.53The same classification is applicable to the hydroxyl groups of GO.Therefore,the oxygen functionalities of GO are of six types,as presented in Figure 2:A ,A ′,B ,B ′,C ,and D .We use various cluster models of GO for oxygen functionalities of these six types (Chart 1).Models 3a ,4a ,and 5a are for GO with the oxygen functionality A .1b ,2b ,4b ,and5b mimic GOs with oxygen functionality A ′.5c -f and 6a are models for GO with functionality B .Both 5g and 5h are models of GO with functionality B ′.Models 6a and 4c are respectively the models of GOs with carbonyl and carboxyl groups.The aromatic domains of 3a ,4a ,and 5a gradually become larger,as do those of 1b ,2b ,4b ,and 5b .These two sets are used to investigate the size effect on the de-epoxidation of GO.The hydroxyl groups of 5c -f gradually move away from the interior to the edge of 5.Therefore,5c -f are used to investigate the edge effect on the dehydroxylation of GO.putational Methods.All calculations were per-formed with the GAUSSIAN 03program.54Geometry optimiza-tions for all stationary points (energy minima and transition states)were done with the M05-2X 55density functional combined with the 6-31G(d)56,57basis set.For all optimized geometries,we did frequency analyses at the same level of theory (M05-2X/6-31G(d))to calculate entropies (S ),enthalpies (H ),and Gibbs free energies (G ).We characterized all transition states as geometries exhibiting only one imaginary frequency,and all energy minima as geometries without imaginary frequencies.For each representative reaction path,we performed an intrinsic reaction coordinate (IRC)calculation at the level of M05-2X/6-31G(d)to verify that the transition states are directed correctly toward the energy minima.We applied a spin-unrestricted M05-2X (UM05-2X)method for radical species of 5c -h ,6,OH radical,and the transition states for dehydroxy-lations of 5c -h and 6a .We used a spin-restricted M05-2X (RM05-2X)method to calculate the other closed-shell singlet species.The transition state for the dehydroxylation of 6a has an open-shell singlet ground state.Therefore,an initial guess with broken symmetry was used for the calculation of this transition state.To obtain an energy in the presence of solvent,we applied the single-point energy calculation using the PCMTABLE 1:Elemental Compositions of GOs before and after Hydrazine and Thermal Reductionreduction processGO (before reduction)CMG (after reduction)ref hydrazine hydride is added to an aqueous GO solution,then the system is kept at 100°C for 1dayC:O:N )2.7:1:0C:O:N )10.3:1:0.6ref 47a GO film is dispersed and stirred in anhydrous hydrazine (98%)for 1week;the resulting hydrazinium graphene suspension is spin-coated onto a Si/SiO 2substrate and thermally annealed at 150°CC:O:N )1.3:1:0C:O:N )7.7:1:1.4(before annealing);C:O:N )12:1:0.7(after annealing)ref 48GO film on Si 3N 4/Si substrate is annealed at 900°C in ultrahigh vacuum for 15minC:O )2.8:1C:O )14.1:1ref 49SCHEME 1:Mechanism Proposed in Reference 47a for the De-epoxidation of GO withHydrazineFigure 1.Schematic illustration of questions addressed in thisarticle.Figure 2.Schematic illustration of oxygen-containing groups in GO:A ,epoxide located at the interior of an aromatic domain of GO;A ′,epoxide located at the edge of an aromatic domain;B ,hydroxyl located at the interior of an aromatic domain;B ′,hydroxyl at the edge of an aromatic domain;C ,carboxyl at the edge of an aromatic domain;and D ,carboxyl at the edge of an aromatic domain.Hydrazine and Thermal Reduction of Graphene Oxide J.Phys.Chem.C,Vol.114,No.2,2010833model 58and the M05-2X/6-31G(d)method for the geometry optimized without solvent.Reportedly,the M05-2X density functional is accurate in calculating bond dissociation energies.55To confirm the accuracy of the M05-2X/6-31G(d)in predicting the reduction kinetics of GO,we calculated the reaction energy profile for the hydrazine reduction of 2b using two methods:M05-2X/6-31G(d)and CCSD(T)59/cc-pVDZ.60The results are depicted in Figure 3.Both methods predict similar reaction energy profiles (Figure 3).Consequently,M05-2X/6-31G(d)is a computationally ef-ficient method with moderate accuracy that is practically feasible for studying the deoxygenation mechanisms of GO with treatment of hydrazine and heat.3.Results and Discussion3.1.Mechanisms of Hydrazine De-epoxidation under Vacuum.We found three reaction routes ;Routes 1,2,and 3;for removal of the epoxide group in GO using hydrazine as a reducing agent under vacuum (Scheme 2).In contrast,we have not found the mechanism of Scheme 1.47a In Route 1of Scheme 2,de-epoxidation occurs in three steps:First,hydrazine (N 2H 4)attacks the carbon of epoxide from the back side of the epoxide ring.This step opens the ring of epoxide and forms int 1.Second,the C -C bond of int 1rotates to bring the hydrazine group (-H 2NNH 2)attached to the opposite side of the oxygen close to the oxygen.Then one H-atom transfers from the hydrazine group to the oxygen of epoxide,forming a hydrazino alcohol int 2.Third,another H of int 2transfers from the hydrazino group (-HNNH 2)to the hydroxyl group (-OH).This gives a water molecule (H 2O),a cis-diazene (cis-N 2H 2),andthe deoxygenated product.In Route 2,the de-epoxidation proceeds in two steps:First,N 2H 4attacks the sp 2-carbon nearest to the epoxide group from the front side of the epoxide ring.One H is transferred from N 2H 4to the epoxide,yielding int .The second step progresses similarly to the third step of Route 1to give H 2O and cis-N 2H 2and finish the deoxygenation.In Route 3,de-epoxidation occurs in three steps.First,N 2H 4attacks the sp 2-carbon located at the meta position of epoxide to give int 1.Second,the -OH and -HNNH 2groups of int 1rotate in aCHART 1:Fragmental Graphene and GO SheetsaaHere,1-6are fragments of graphene.3a ,4a ,and 5a have epoxides on the basal planes of graphene sheets.1b ,2b ,4b ,and 5b have epoxides at the edges of aromatic domains.6a and 5c -h have hydroxyl groups.Drawn as black circles in 5c -h are the positions to which the hydroxyl groups are attached.Both 4c and 6b have carboxyl and carbonyl groups.Hydrogen atoms are omitted in the figure forclarity.Figure 3.Reaction energy profiles for the hydrazine de-epoxidation of 2b via Route 2(Scheme 2),calculated using M05-2X/6-31G(d)and CCSD(T)/cc-pVDZ.In both calculations,geometries of the stationary points were optimized at the level of M05-2X/6-31G(d).Geometries between stationary points were optimized by following the intrinsic reaction coordinates with a step size of 0.015amu 1/2·Bohr,using the same level of theory.The energy profile at the level of CCSD(T)/cc-pVDZ was obtained with single-point energy calculations based on the M05-2X/6-31G(d)geometries.834J.Phys.Chem.C,Vol.114,No.2,2010Gao et al.disrotatory mode,converting int 1to int 2.The third step is similar to the third step of Route 1.The hydrazine de-epoxidation of 1b follows Route 1,whereas those of 3a ,4a ,5a ,2b ,4b ,and 5b follow either Route 2or Route 3.Figure 4presents the reaction energy profiles for the reductions of 5a and 5b .Figure 5portrays the three-dimensional atomic structures for the stationary points involved in the hydrazine reduction of 5a .Table 2presents the energies of the stationary points in the reductions of 3a ,4a ,2b ,4b ,and 1b .Table 2shows that for the hydrazine de-epoxidation of 3a via Route 2under vacuum,the second step (second H-transfer)is the rate-determining step.This step has a Gibbs free-energy barrier of 51.1kcal/mol at room temperature (G q 25°C )45.3-(-5.8))51.1kcal/mol)(Table 2).For the de-epoxidation of 3a via Route 3under vacuum,the first step (the first H-transfer)is rate determining,with G q 25°C )48.3kcal/mol (Table 2).Therefore,Route 3is slightly more efficient than Route 2for the hydrazine reduction of 3a .In the hydrazine de-epoxidation of 4a (5a ),the first step (first H-transfer)is rate determining,irrespective of via Route 2or Route 3.However,the de-epoxidation of 4a (5a )via Route 2has G q 25°C of 41.2(34.9)kcal/mol.This barrier is lower than the corresponding barrier in Route 3,which is 49.3(38.0)kcal/mol (Table 2and Figure 4).Therefore,Route 2is kinetically more favorable than Route 3in the de-epoxidation of 4a (5a )with hydrazine.These results suggest that Route 3dominates hydrazine reductions when the epoxides being attacked are located at the interior of a small aromatic domain,but Route 2is expected to prevail if those interior epoxides belong to a large aromatic domain.Table 2and Figure 4show that hydrazine reductions of 3a ,4a ,and 5a are exothermic.The enthalpy changes (∆H )for 3a ,4a ,and 5a are respectively -33.6,-46.1,and -41.5kcal/mol.The corresponding changes in the Gibbs free energy at room temperature (∆G 25°C );given respectively as -4.1,-57.0,and -53.2kcal/mol ;are even more negative.Meanwhile,the intermediates involved in these reductions,the hydrazino alcohols,are thermally much less stable than the corresponding reduction products.The hydrazine de-epoxidations of 3a ,4a ,and 5a are therefore thermodynamically spontaneous processes.However,the reaction energy barriers (G q 25°C )for these pro-cesses are respectively 48.3,41.2,and 34.9kcal/mol.The energy barriers for 3a ,4a ,and 5a suggest that the hydrazine reduction of epoxide becomes more favorable if it belongs to a larger aromatic domain.Consequently,epoxides at the interior of an aromatic domain of GO will be removed by hydrazine at reaction temperatures of 100-150°C.47a,48Table 2and Figure 4show that the first H-transfer is the rate-determining step in the hydrazine de-epoxidations of 4b and 5b via Route 3,whereas the second H-transfer is rate determining in the other de-epoxidations.The G q 25°C values for the de-epoxidations of 2b ,4b ,and 5b via Route 2are 60.7,68.8,and 71.7kcal/mol,respectively;those via Route 3are 44.0,63.2,and 70.2kcal/mol,respectively.Therefore,the kinetically favorable pathways for hydrazine reductions of 2b ,4b ,and 5b should follow respectively Routes 3,2,and 2.In contrast,the de-epoxidation of 1b with hydrazine exclusively follows Route 1with G q 25°C )83.5kcal/mol (Table 2).Hydrazine de-epoxidations of 1b ,2b ,4b ,and 5b exhibit features that differ from those of 3a ,4a ,and 5a .In fact,the ∆G 25°C values for these are -2.3to -34.6kcal/mol and are less negative than those for 3a ,4a ,and 5a (Table 2and Figure 4).The de-epoxidations of 1b ,4b ,and 5b involve stable hydrazino alcohol intermediates:int 2for 1b ,int for 4b ,and int for 5b .These intermediates have Gibbs free energies of formation of -25.2,-21.6,and -29.8kcal/mol at room temperature,respectively,which are even lower than those forSCHEME 2:GO Reduction MechanismsaaRoutes 1-3and 2′represent the mechanisms for the hydrazine de-epoxidation of GO.Routes 4and 5refer to the mechanism for the thermal de-hydroxylation of GO.Routes 6and 7respectively show the mechanisms for thermal de-carbonylation and thermal de-carboxylation of GO.Hydrazine and Thermal Reduction of Graphene Oxide J.Phys.Chem.C,Vol.114,No.2,2010835the corresponding reduction products (i.e.,-0.4,-20.6,and -22.6kcal/mol)(Table 2and Figure 4).Therefore,the hydrazine de-epoxidation for 1b ,4b ,and 5b will stop with the formation of a hydrazino alcohol.The reaction energy barriers (G q 25°C )for the de-epoxidations of 2b ,4b ,and 5b are 44.0,62.5,and 69.2kcal/mol,respectively,showing an increasingbehaviorFigure 4.Reaction energy profiles for hydrazine de-epoxidations of 5a and 5b under vacuum calculated at the M05-2X/6-31G(d)level of theory.Values in normal and italic typefaces are respectively relative enthalpies (H rel ,in kcal/mol)and relative Gibbs free energies (G rel ,in kcal/mol)at room temperature (25°C).Figure 5.Local atomic structures for stationary points involved in hydrazine de-epoxidations of 5a via Routes 2and 3.836J.Phys.Chem.C,Vol.114,No.2,2010Gao et al.concomitantly with increased size of aromatic domain.Conse-quently,hydrazine reductions of epoxides located at the edgesof GO become increasingly difficult as the aromatic domain size increases.3.2.Mechanisms of Thermal Dehydroxylation under Vacuum.We have found no reaction route corresponding to the reduction of the hydroxyl group of GO with hydrazine as a reducing agent.Hydrazine plays a minor role,if any,in the dehydroxylation of GO.Instead,we have located two reduction routes without hydrazine:Routes 4and 5(Scheme 2).In Route 4,the hydroxyl reduction takes place in two steps and yields a monoxide (CO)and a graphene sheet with a vacancy.By contrast,in Route 5,the hydroxyl group directly leaves the graphene sheet,producing an OH radical and a graphene radical.Xu et al.61studied the dissociative adsorption of an OH radical on graphite surfaces.They reported similar routes for the reaction of an OH radical with their model graphite.61Figure 6a depicts the reaction energy profile for reductions of 6a via Routes 4and 5.They are respectively both endother-mic with ∆H )99.0and 30.1kcal/mol.The corresponding ∆G 25°C and G q 25°C values are respectively 85.5and 18.1kcal/mol,and 89.9and 27.7kcal/mol.Given the significantly higher values in G q 25°C and ∆G 25°C ,Route 4is expected to be much less competitive than Route 5in the thermal reduction of hydroxyl groups of GO,which accords with the results of Xu et al.61We will therefore not consider Route 4in the following discussions.We studied the dehydroxylations of 5c -h via Route 5to investigate the manner in which dissociation energies depend on the positions of hydroxyl groups of GO.The hydroxyl groups of 5c -h increasingly move their positions away from the interior toward the edges of 5(Chart 1).The reaction energy profiles are depicted in Figure 6b.The dehydroxylation of 5c (5d -f )via Route 5has a transition state with G q 25°C of approximately 13.8kcal/mol;the dehydroxylation of 5g (5h )via Route 5involves no transition state (Figure 6b).Figure 6b presents that all dehydroxylations of 5c -h are endothermic with ∆H values of 7.2-35.6kcal/mol.In addition,∆G 25°C values for 5c -h are respectively -3.3,-3.5,-3.1,-2.7,25.8,and 16.5kcal/mol.A negative ∆G 25°C and a low G q 25°C for 5c -e suggest that a single hydroxyl group attached to the interior aromatic domain of 5(i.e.,5c -f )is not stable and is subject to dissociation at room temperature.In contrast,a positive ∆G 25°C for 5g and 5h suggests that a hydroxyl attached at the edge of 5is stable at room temperature.Hydroxyls attached to the inner aromatic domains of GO are therefore expected to dissociate or migrate to the edges of aromatic domains.We further investigate how to reduce hydroxyls at the edges of aromatic domains of GO (see below).3.3.Mechanisms of Thermal Decarbonylation and De-carboxylation under Vacuum.We have not found reaction routes for which the carbonyl and carboxyl groups of GO are removed with hydrazine treatment.As in dehydroxylation,hydrazine will not play an important role in the decarbonylationTABLE 2:Reaction Enthalpies (kcal/mol)and Gibbs Free Energies (25°C,in Parentheses,kcal/mol)for Stationary Points Involved in Hydrazine De-epoxidations of 3a,4a,1b,2b,and 4b under Vacuum at the M05-2X/6-31G(d)Level of Theory ade-epoxidation via Route 2de-epoxidation via Route 3reactantts 1int ts 2ts 1int 1ts 2int 2ts 3product3a +N 2H 40.0(0.0)29.1(42.4)-18.1(-5.8)33.1(45.3)35.8(48.3)-15.2(-2.5)-8.0(4.9)-17.6(-5.6)17.1(29.6)-33.6(-44.1)4a +N 2H 40.0(0.0)27.1(41.2)-8.5(4.9)9.6(22.4)35.6(49.3) 2.2(15.5)10.7(24.3)8.0(20.8)11.0(23.8)-46.1(-57.0)2b +N 2H 40.0(0.0)20.0(33.6)-28.8(-16.7)32.2(44.1)25.9(38.5)-24.3(-11.9)-20.2(-7.7)-25.8(-13.9)17.8(30.1)-22.1(-33.1)4b +N 2H 40.0(0.0)35.4(47.8)-34.4(-21.6)43.9(58.9)49.8(62.5)0.3(13.3)9.5(22.5) 3.3(15.9)21.0(33.9)-9.5(-20.6)de-epoxidation via Route 1reactantts 1int 1ts 2int 2ts 3product 1b +N 2H 40.0(0.0)36.0(47.6)33.1(44.7)35.0(47.4)-37.2(-25.2)46.5(58.4)9.7(-0.4)aFor definitions of 3a ,4a ,1b ,2b ,and 4b ,see Chart 1;for definitions of Routes 1-3,see Scheme2.Figure 6.Reaction energy profiles for the dehydroxylation of (a)6a and (b)5c -h under vacuum obtained from the M05-2X/6-31G(d)level of theory.Values in normal and italic print respectively show relative enthalpies (H rel ,in kcal/mol)and relative Gibbs free energies (G rel ,in kcal/mol)at room temperature (25°C).The inset presents schematic structures of pro (6a )and ts (6a ).Hydrazine and Thermal Reduction of Graphene Oxide J.Phys.Chem.C,Vol.114,No.2,2010837or decarboxylation of GO.However,we found reaction routes without hydrazine,which correspond to thermal decarbonylation and decarboxylation of GO under vacuum:Routes 6and 7(Scheme 2).Both routes involve one-step deoxygenation with a transition state.Figure 7a presents the reaction energy profile for the decarbonylation of 6b via Route 6.The thermal decarbonylation under vacuum is endothermic (∆H )56.2kcal/mol).The corresponding ∆G 25°C and G q 25°C values are 44.5and 133.0kcal/mol,respectively,meaning that the decarbonylation of 6b via Route 6does not occur spontaneously at room temperature.Furthermore,hydrazine can add to the C d O double bond of 6b ,forming a hydrazino alcohol (Figure S1of the Supporting Information).This addition reaction has a positive ∆G 25°C as well (∆G 25°C )6.7kcal/mol)and is not spontaneous.This addition reaction involves a decrease in entropy (∆S <0).Therefore,the increased reaction temperature will make ∆G more positive.This addition reaction will not occur spontane-ously at a higher temperature either.Figure 7b shows the reaction energy profile for the vacuum decarboxylation of 4c via Route 7.Actually,∆G 25°C )-14.3kcal/mol and G q 25°C )65.7kcal/mol for thermal decarboxylation.This decarboxylation is expected to occur slowly at room temperature.These results suggest that the carbonyl groups of GO will not be removed spontaneously,but the carboxyl groups are expected to be reduced slowly at temperatures of 100-150°C.47a,483.4.Temperature Effect on GO Reduction.Reports of experimental studies have described the importance of temper-ature in the deoxygenation of GO.A recent study by Yang et al.49has demonstrated that the increase of temperature improves the efficiency of thermal reduction considerably.Thermal annealing of GOs at 500,700,and 900°C respectively yielded CMGs with C:O ratios of 8.9,13.2,and 14.1.49Tung et al.48and Stankovich et al.47a also reported the importance of thermal annealing.As described above,epoxide (hydroxyl)groups located at the interior of an aromatic domain of GO can be eliminated by using hydrazine (thermally annealing)with relative ease,but those located at the edges of GO are difficult to remove (see Sections 3.1and 3.2).Therefore,epoxides and hydroxyls at the edges of aromatic domain deserve special attention.Figure 8a presents the reaction energy profile for the hydrazine de-epoxidation of 5b via Route 2for various temperatures.When the temperature increases from -175to 1125°C,∆G decreases from -10to -60kcal/mol.The value of G 1q for the first step of reaction increases from 40to 100kcal/mol (Figure 8a).That increase in G 1q results from the fact that the first step in the reaction relies upon the capture of a hydrazine molecule on the moiety 5b (∆S <0).An increased temperature reduces the probability for such capturing;consequently,it will not improve the efficiency of hydrazine de-epoxidation.Figure 8b presents the reaction energy profile for the thermal dehydroxylation of 5h via Route 5at various temperatures.When the temperature increases,the ∆G decreases from positive to negative (Figure 8b).The critical temperature (T c ),defined as the point at ∆G )0,is 650°C,showing that raising the temperature will make the dehydroxylation of GO spontaneous.When the temperature is greater than 650°C,49even the hydroxyls attached to the edges of GO can be readily removed.As for the thermal decarboxylation of 4c via Route 7,an increase in temperature will reduce ∆G without increasing the reaction energy barrier.Raising temperatures is therefore going to facilitate the thermal decarboxylation of GO as well.Although a rise in temperature is also expected to expedite thermal decarbonylation of 6b via Route 6,the corresponding T c is as high as 1730°C.After thermal annealing at about 1000°C,49the GO’s carboxyl groups will be removed,but GO’s carbonyl groups will remain.Given the opposite temperature dependences of hydrazine de-epoxidation and thermal dehydroxylation/decarboxylation,it is necessary to perform these two typesofFigure 7.Reaction energy profiles for (a)decarbonylation of 6b and (b)decarboxylation of 4c under vacuum.Calculation used the M05-2X/6-31G(d)level of theory.Values in normal and italic print typefaces respectively depict relative enthalpies (H rel ,in kcal/mol)and relative Gibbs free energies (G rel ,in kcal/mol)at room temperature (25°C).Schematic structures of stationary points are also shown.838J.Phys.Chem.C,Vol.114,No.2,2010Gao et al.。